Gas Phase NMR / Edition 1 available in Hardcover
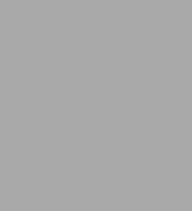
Gas Phase NMR / Edition 1
- ISBN-10:
- 178262161X
- ISBN-13:
- 9781782621614
- Pub. Date:
- 02/18/2016
- Publisher:
- RSC
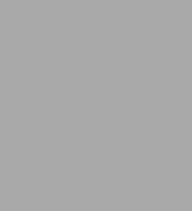
Gas Phase NMR / Edition 1
Hardcover
Buy New
$251.00-
SHIP THIS ITEM— Not Eligible for Free Shipping
Overview
The progress of research in these fields is enormous and has rapidly changed our knowledge and understanding of molecular parameters in NMR spectroscopy. For example, accurate studies of the shielding for isolated molecules allow the exact determination of nuclear magnetic dipole moments, the calculated values of spectral parameters can be verified by precise gas-phase NMR measurements, and the application of hyperpolarized noble gases provides excellent MRI pictures of lungs. Aimed at graduates and researchers in spectroscopy, analytical chemistry and those researching the applications of NMR in medicine, this book presents the connections between sophisticated experiments, the theory of magnetic parameters and the exploration of new methods in practice.
Product Details
ISBN-13: | 9781782621614 |
---|---|
Publisher: | RSC |
Publication date: | 02/18/2016 |
Series: | New Developments in NMR , #6 |
Pages: | 418 |
Product dimensions: | 6.15(w) x 9.20(h) x (d) |
Read an Excerpt
Gas Phase NMR
By Karol Jackowski, Michal Jaszunski
The Royal Society of Chemistry
Copyright © 2016 The Royal Society of ChemistryAll rights reserved.
ISBN: 978-1-78262-161-4
CHAPTER 1
Fundamental Intramolecular and Intermolecular Information from NMR in the Gas Phase
CYNTHIA J. JAMESON
University of Illinois at Chicago, USA Email: cjjames@uic.edu
1.1 Why Should One Do Gas Phase NMR Measurements?
In the gas phase we have a well-defined homogeneous physical system, and the theory for dilute gas behavior is in an advanced stage. In dilute gases, we can expand the molecular electronic property (e.g., nuclear magnetic shielding, J coupling, nuclear quadrupole coupling) in a virial expansion, in which the property virial coefficients can be expressed theoretically in closed form and can be obtained unequivocally experimentally in the binary interaction limit. These experimentally measured quantities depend on two quantum-mechanical mathematical surfaces: the shielding, or J, or electric field gradient (efg) at the nucleus as a function of intermolecular nuclear coordinates and the weak intermolecular interaction potential energy surfaces that are also a function of the same intermolecular nuclear coordinates. Furthermore, we can extrapolate the measured NMR data (shielding, J, efg) to the zero-density limit to obtain these electronic properties for the isolated molecule, that are much more closely related to quantum-mechanical calculations than quantities measured in condensed phases. To validate theoretical methods, it is always preferable to benchmark the results by comparing them with available experimental data, preferably for isolated molecules. Extrapolation to this limit is only possible for gas phase measurements. Here too, the temperature dependence of the electronic property at the zero-density limit is a function of two quantum-mechanical mathematical surfaces: the shielding (or J or efg) as a function of intramolecular nuclear coordinates and the intramolecular potential energy surface that are also a function of the same coordinates. The latter is commonly characterized by specifying the derivatives at the equilibrium intramolecular configuration, namely the quadratic, cubic, quartic force constants. The shielding is particularly sensitive to the anharmonicity of the intramolecular potential surface. Thus, gas phase NMR data for shielding, J, and efg provide stringent tests of theoretical descriptions of both the quantum-mechanical electronic property surfaces and also the potential energy surfaces over which they are averaged, to yield the temperature-dependent experimental data (property virial coefficients and zero-density limiting values) that are available only in the dilute gas phase. In addition to temperature, another variable, isotopic masses of neighboring (and observed) nuclei, can affect the measured data, given the same electronic property surfaces and the same potential energy surfaces; thus, isotope effects provide an independent test of these quantum-mechanical surfaces. While these observations and their interpretation are of specific interest to NMR spectroscopists, they are of more general interest as prototypes of rovibrational averaging and intermolecular effects on molecular electronic properties. Fortunately, it is possible in NMR spectroscopy to make very precise measurements of quantities that are very sensitive to changes in electronic environment, nuclear magnetic shielding and J, molecular electronic properties that are sensitive indices of the chemical bond and that vary with nuclear displacements from the equilibrium molecular configuration, leading to changes in resonance frequencies that are amenable to highly precise measurements under precisely controlled constant temperature conditions over a wide range of temperatures. Thus, gas phase measurements in NMR provide valuable tests of quantum-mechanically calculated molecular electronic property surfaces. Indeed, the dihedral-angle dependence of three-bond J coupling by Martin Karplus (known to NMR spectroscopists as the Karplus equation) was the earliest (1959) example of an experimentally testable quantum-mechanically calculated property surface. An important disadvantage of gas phase NMR, however, is that only the isotropic values of the NMR tensor quantities can be obtained.
For the same reasons, NMR spectra of dilute gases provide thermodynamic and kinetic information that are important from a theoretical point of view. The gas phase allows the separation of intramolecular and environmental effects on the energy requirements for molecular processes. Gas phase NMR data provide the free energy barriers for conformational changes, from which torsional parameters for molecular dynamics (MD) force fields are obtained. Furthermore, pressure can be used as an experimental variable in gas phase studies; rate constants are both temperature - and pressure-dependent. Use of dynamic gas phase NMR techniques permits the complete characterization of rate processes within both temperature and pressure ranges, allowing the kinetics of chemical rate processes to be investigated in both the unimolecular and bimolecular regimes. Information about internal vibrational redistribution and collisional energy transfer in kinetic processes is obtained from these NMR studies. Thus, conformational dynamics can be characterized under well-defined limiting conditions in the gas phase, free energy barriers can be obtained, and theoretical interpretation of results using well-established methods can provide detailed interpretation. A collateral experimental advantage is the rapid spin–lattice relaxation that facilitates multiple acquisitions; 13C relaxation times are at least two orders of magnitude shorter in the gas phase for some systems than in condensed phases. In the gas phase, we can measure spin–lattice relaxation rates that are of fundamental interest in their own right. The rates are resolvable into well-defined mechanisms via measurements as a function of field, of temperature, of density. Furthermore, in the gas phase, each relaxation mechanism is capable of being theoretically calculated via classical trajectory calculations in the binary collision limit, yielding well-defined relaxation cross-sections that are well-established descriptions of fundamental dynamic molecular events, such as transfer of rotational angular momentum and molecular reorientation, that provide valuable stringent tests of the anharmonicity of intermolecular potential surfaces.
Reviews of gas phase NMR studies include some of these measurements of shielding and spin–spin coupling, spin-relaxation studies, and conformational changes, that provide more detailed information and references to original literature not included in the present overview.
1.2 The Effect of Intermolecular Interactions on NMR Chemical Shifts
Buckingham and Pople proposed in 1956 that any electromagnetic properties of gases be expanded in a virial expansion. For nuclear magnetic shielding in a pure gas
[FORMULA OMITTED] (1.1)
For a nucleus X in molecule A in a dilute mixture of gases A and B,
[FORMULA OMITTED] (1.2)
In the gas of pure A, this expansion permits the study of the intermolecular contributions by investigating the temperature dependence of the density coefficient of nuclear shielding σ1AA(T), i.e., the slope ofσX in A (T, ρA) as a function of density ρA, in the limit of linear behavior. At the same time this permits the study of the intramolecular contributions, I σ0X in A (T), by in the temperature dependence of nuclear shielding in the limit of zero gas density. This term arises from the variation with temperature of the populations of the rotational and vibrational states, each one of which has a different characteristic average shielding. This is mathematically equivalent to extrapolation to a pressure that is low enough that collisional deformation of the molecules no longer contributes to σ however there are still sufficient collisions to provide averaging over the rovibrational states of the molecule. The quantity σ0X in A(T), is the shielding in a molecule free of intermolecular interactions and therefore equivalent to an isolated molecule. From experimental measurements in the linear-density regime, each observed shift can be corrected for the intermolecular contributions σ1(T) ρ so that the remainder, σ0(T) - σ1(300 K)], is obtained.
In gas mixtures with low mole fraction of A in B, subtraction of the accurately determined AA contributions permits the determination of σ1AB(T). The quantity σ1AA(T) is a measure of the effects on nuclear magnetic shielding of X in molecule A from binary collisions of A with another A molecule and σ1AB(T) is a measure of the effects from binary collisions of A with molecule B. The excess intermolecular property, σ2(T)ρ2 + σ3(T)ρ3 ···, has been investigated in some cases, for example for 129Xe in Xe gas, where collectively this has been found to be opposite in sign to σ1(T)ρ There are experimental indications that this is true for other nuclei in other gases as well, for example, 19F in H2C=CF2. Our main focus in this section is on the density coefficient of NMR properties in the limit of zero density, that is, the second virial coefficient of shielding. There is an experimental quantity that also has a linear density dependence, the bulk susceptibility contribution to the observed chemical shift, that is the same amount for all nuclei in the sample, that is an artifact of the sample shape and vanishes for spherical samples. It is understood in this section that experimental values of the second virial coefficient will have been corrected for the sample shape contribution, since we are interested in the true shielding response that arises from binary intermolecular interactions. This susceptibility correction limits the precision of experimental values, but is of consequence only in those cases where the true second virial coefficient of shielding is smaller than this correction.
1.2.1 The Density Coefficient of the Chemical Shift
The first observation of the density coefficient of the chemical shift in a gas was by Streever and Carr in 1961 for 129Xe in xenon gas, followed soon thereafter by Gordon and Dailey for 1H in CH4 and C2H6, and in 1962 by Raynes, Buckingham, and Bernstein for 1H in H2S, CH4, and C2H6 and for HCl in various gas mixtures. Measurements of the second virial coefficient of nuclear magnetic shielding have been carried out for a variety of nuclei; the largest values are those for 129Xe in Xe atom interacting with another rare gas atom or molecule. Second virial coefficients of shielding of other nuclei, for example, of 1H in HCl, HBr, HCN, NH3, C2H4, C2H6, C3H8, of 11B in BF3, of 13C in CO, CO2, HCN, CH4, of 15N in N2, NNO, NH3, of 17O in CO, CO2, NNO, OCS, of 31P in PH3, PF3, PF5, POF3, of 19F in a large number of molecules (ref. 30, 31 and references therein) have been investigated as a function of temperature. Recent additions to these include all the nuclei in propene, in cyclopropane, in CHF3, in CH2 F2, in SO2 and SO3, in SiF4, in CH3OH, in (CH3)2O, in CH3NH2, in CH3CN, and in (CH3)4Sn. The linear-density coefficient of 13C in benzene, acetylene, and CH3Br, and of 33S in SF6 have also been studied.
An advantage of gas phase studies in the linear-density regime is that the intermolecular effects on shielding can be expressed in closed mathematical form, just as derived in general for any electromagnetic molecular property by Buckingham and Pople. For Xe interacting with CF4, for example,
[MATHEMATICAL EXPRESSION OMITTED] (1.3)
The theoretically expected behavior of σ1(T) in rare gas systems over a wide temperature range has been shown to be negative (deshielding with increasing density), increasing in magnitude with increasing temperature, then switching over and decreasing in magnitude with increasing temperature (see Figure 6 in Ref. 48). For rare gas atoms, it has been found that the sign of σ1(T) is indeed negative at all temperatures, that is, the nucleus becomes more deshielded with increasing density. For nuclei of end atoms in a molecule, the sign of σ1 is generally negative at all temperatures. Known exceptions are σ1(15N) in CH3CN and HCN, in which intermolecular interactions involve the lone pair and thereby affect n [right arrow] π* contributions to the 15N shielding toward less deshielding (such as that which accompanies a blue shift in the n [right arrow] π* transition energy). For more centrally located nuclei in a molecule, the general behavior, sign, or temperature dependence has not been calculated, but magnitudes are expected to be smaller than for end atoms. This behavior is not generalizable since, unlike the end atoms which experience intermolecular effects directly, a nucleus in centrally located atoms (except in linear molecules) can only experience intermolecular effects indirectly through chemical bonds, hence is dependent on the specific molecular structure surrounding the observed nucleus. In those cases where the temperature dependence has been measured over a wide range of temperatures, it has been found that the magnitude of σ1(T) generally decreases with increasing temperature; exceptions are 129Xe in CO and 129Xe in N2, which are not anomalous behavior since σ1(T) has been theoretically predicted to turn around to decreasing magnitudes at much lower temperatures, in general. The magnitudes and signs of very small σ1 for less exposed nuclei such as 33S in SF6, 13C in CH4, 29Si in SiF4 are difficult to obtain accurately in experiments because they are generally small and the experimental density coefficient is thus dominated by bulk susceptibility contributions for non-spherical sample shapes.
There is clear evidence of a nuclear site effect in the same molecule, with more exposed nuclei having larger values of σ1 than less exposed ones. In a clear example, the three chemically inequivalent F nuclei in XFC = CF2, Fgem, Ftrans, Fcis, have different distances from the center of mass of the molecule, and these relative distances change as X goes from light to heavy mass. Within the freely rotating molecule, the relative exposure of each of the three 19F sites to intermolecular interactions are reflected in their respective values of σ1 in XFC = CF2 molecules (X = H, F, Cl, Br, I), completely consistent with the relative distance of each of Fgem, Ftrans, Fcis from the center of mass as the latter changes systematically from X = H, to F, to Cl, to Br, to I. A more transparent and elegant example of the nuclear site effect was demonstrated experimentally by Beckett and Carr in the density dependence (in HD gas of varying density with a small amount of D2) of the isotope shift [σ(D2) - σ(HD)] = a + bρ. Here, a = [sigma0(D2) - σ 0 (HD)] is the isotope shift extrapolated to the zero-density limit. Their observation that the density coefficient of the isotope effect, b < 0 means that |σ1 (D2)|> | σ 1 (HD)| since all σ1 is known to be negative. The greater magnitude of |σ1 (D2)| arises from the more exposed deuterium nucleus in D2 (R/2 from the center of mass in this isotopomer) compared to HD where the D is R/3 from the center of mass. The density coefficient b can be calculated from the site factors, as shown in ref. 50. Thus, the nuclear site effect gives rise to the observed density dependence of the isotope shift in the Beckett and Carr experiments.
In some cases, intermolecular effects on shielding have been measured from very low density gas to the liquid phase in a single experiment. It is especially interesting when both gas and liquid are observed in the same sample tube as a function of temperature. The difference in chemical shift between the liquid and the overhead vapor should approach zero in the limit of the critical temperature. Indeed, this behavior has been observed for 19F in a large number of compounds (see for example ref. 50).
1.2.2 The Intermolecular Shielding Function
The first ab initio shielding function calculated for a rare gas pair [σ (R) - σ(∞)] was that for Ar–Ar; these were restricted Hartree–Fock (RHF) calculations of σ(R) from large separations all the way to an internuclear distance of 1 Å, or 0.30 times r0 (Ar–Ar). In this specific case the united atom in the correlation diagram of the two Ar atoms is a closed shell ground state (Kr) so that it is possible to extrapolate the shielding function all the way to the united atom, and thereby observe the general shape of an intermolecular shielding function. The intermolecular shielding function for Xe interacting with rare gas atoms Ne, Ar, Kr, Xe has been calculated with very large basis sets at various levels of accuracy. With the inclusion of relativistic corrections, the final agreement with the experimental temperature dependence is almost within experimental error. The shielding functions all change steeply in the vicinity of r0 of the potential energy surface (PES).
(Continues...)
Excerpted from Gas Phase NMR by Karol Jackowski, Michal Jaszunski. Copyright © 2016 The Royal Society of Chemistry. Excerpted by permission of The Royal Society of Chemistry.
All rights reserved. No part of this excerpt may be reproduced or reprinted without permission in writing from the publisher.
Excerpts are provided by Dial-A-Book Inc. solely for the personal use of visitors to this web site.