On the night before his fatal duel, 20-year-old Evariste Galois (1811-32) scribbled madly in his notebook, so frantically that he wrote in the margins, "I have no time. I have no time." This impetuous youth did not live to witness his triumph, but posterity would recognize his elegant proof as the conclusion of a 4,000-year mathematical quest. In The Equation that Couldn't Be Solved, Mario Livio tells the story of the search for the quintic equation and the birth of group theory, the "language" that describes symmetry.
What do Bach's compositions, Rubik's Cube, the way we choose our mates, and the physics of subatomic particles have in common? All are governed by the laws of symmetry, which elegantly unify scientific and artistic principles. Yet the mathematical language of symmetry-known as group theory-did not emerge from the study of symmetry at all, but from an equation that couldn't be solved.
For thousands of years mathematicians solved progressively more difficult algebraic equations, until they encountered the quintic equation, which resisted solution for three centuries. Working independently, two great prodigies ultimately proved that the quintic cannot be solved by a simple formula. These geniuses, a Norwegian named Niels Henrik Abel and a romantic Frenchman named Évariste Galois, both died tragically young. Their incredible labor, however, produced the origins of group theory.
The first extensive, popular account of the mathematics of symmetry and order, The Equation That Couldn't Be Solved is told not through abstract formulas but in a beautifully written and dramatic account of the lives and work of some of the greatest and most intriguing mathematicians in history.
What do Bach's compositions, Rubik's Cube, the way we choose our mates, and the physics of subatomic particles have in common? All are governed by the laws of symmetry, which elegantly unify scientific and artistic principles. Yet the mathematical language of symmetry-known as group theory-did not emerge from the study of symmetry at all, but from an equation that couldn't be solved.
For thousands of years mathematicians solved progressively more difficult algebraic equations, until they encountered the quintic equation, which resisted solution for three centuries. Working independently, two great prodigies ultimately proved that the quintic cannot be solved by a simple formula. These geniuses, a Norwegian named Niels Henrik Abel and a romantic Frenchman named Évariste Galois, both died tragically young. Their incredible labor, however, produced the origins of group theory.
The first extensive, popular account of the mathematics of symmetry and order, The Equation That Couldn't Be Solved is told not through abstract formulas but in a beautifully written and dramatic account of the lives and work of some of the greatest and most intriguing mathematicians in history.
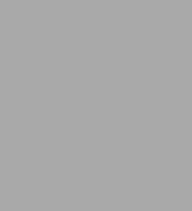
The Equation That Couldn't Be Solved: How Mathematical Genius Discovered the Language of Symmetry
Narrated by Tom Parks
Mario LivioUnabridged — 11 hours, 45 minutes
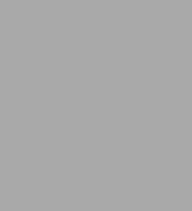
The Equation That Couldn't Be Solved: How Mathematical Genius Discovered the Language of Symmetry
Narrated by Tom Parks
Mario LivioUnabridged — 11 hours, 45 minutes
Overview
What do Bach's compositions, Rubik's Cube, the way we choose our mates, and the physics of subatomic particles have in common? All are governed by the laws of symmetry, which elegantly unify scientific and artistic principles. Yet the mathematical language of symmetry-known as group theory-did not emerge from the study of symmetry at all, but from an equation that couldn't be solved.
For thousands of years mathematicians solved progressively more difficult algebraic equations, until they encountered the quintic equation, which resisted solution for three centuries. Working independently, two great prodigies ultimately proved that the quintic cannot be solved by a simple formula. These geniuses, a Norwegian named Niels Henrik Abel and a romantic Frenchman named Évariste Galois, both died tragically young. Their incredible labor, however, produced the origins of group theory.
The first extensive, popular account of the mathematics of symmetry and order, The Equation That Couldn't Be Solved is told not through abstract formulas but in a beautifully written and dramatic account of the lives and work of some of the greatest and most intriguing mathematicians in history.
Editorial Reviews
The idea of symmetry has been heavily deployed in recent science popularizations to introduce advanced subjects in math and physics. This approach usually backfires-mathematical symmetry is much too difficult for most laypeople to understand. But this engaging treatise soft-pedals it in a crowd-pleasing way. The title's formula is the "quintic" equation (involving x raised to the fifth power), the analysis of which gave rise to "group theory," the mathematical apparatus scientists use to explore symmetry. Inevitably, the author's attempts to explain group theory and its applications in particle physics and string theory to a general audience fall sadly short, so readers will just have to take his word for the Mozartean beauty of it all. Fortunately, astrophysicist Livio (The Golden Ratio) keeps the hard stuff to a minimum, concentrating instead on interesting digressions into human interest (e.g., the founder of group theory, Evariste Galois, was a revolutionary firebrand who died in 1832 at age 20 in a duel over "an infamous coquette"), pop psychology (women have more orgasms when their partners have symmetrical faces), strategies for finding a soul mate and some easy math puzzles readers might actually solve. The result is a somewhat shapeless but intriguing excursion. Photos. Agent, Susan Rabiner. 50,000 first printing; 9-city author tour. (Sept.) Copyright 2005 Reed Business Information.
Livio (former head, science division, Hubble Space Telescope Science Inst., Johns Hopkins Univ.) has written a summary of the origins of group theory and symmetry for lay readers. Taking the approach of his earlier books (The Golden Ratio; The Accelerating Universe), he attempts to bring to nonmathematicians a broad discussion of group theory and aesthetics. No other book covers such a wide range of topics: biographical information on Niels Henrik Abel and Evariste Galois, introductory concepts and problems in group theory, and applications of group theory to broader disciplines-all in one volume. The generously illustrated text has many visual aids and photos, as well as detailed chapter notes with lists of recommended literature sources and a Galois family tree. For the mathematically inclined, Livio also includes more fully explained problems in a series of appendixes. Highly recommended for large public and academic libraries. [See Prepub Alert, LJ 5/15/05.]-Elizabeth Brown, Binghamton Univ. Libs., NY Copyright 2005 Reed Business Information.
Evolution favors symmetry. So do people. So does just about everything in the universe. Astrophysicist Livio (The Golden Ratio, 2002, etc.), no slouch at mathematics himself, crafts an entertaining exploration of how the laws of symmetry have shaped our chaotic little world, and how they inform our appreciation of art and music. One of his great heroes is someone whom mathematicians with a historical bent know well: the French wunderkind Evariste Galois, generally held to be one of the great minds in a field dominated by great minds and the progenitor of what is now called group theory. Galois (1811-32) was a brilliantly troubled kid who loved mathematics, which returned the favor, and a woman who did not. The young genius died in a duel whose occasion has long mystified historians. His last, supremely memorable, words were: "Don't cry, I need all my courage to die at twenty." Before he died, however, Galois impacted the course of history. Among other accomplishments, he formed a new branch of algebra known as Galois theory. Livio's history is elegant but, suffice it to say, not for the innumerate or the scientifically faint of heart: it helps to know something of quadratic equations and other high-order concepts that would have been second nature to Galois but are harder going for us lesser souls. Galois's research, Livio writes, helped turn other scientists to thinking about symmetry, which led to Einstein and quantum theory and other wonders of the modern age. It's a complicated tale, with learned asides on the nature of creativity and, in the bargain, a convincing argument many years after the fact concerning the identity of Galois's killer. A lively companion to Bulent Atalay's Mathand the Mona Lisa (2004), John Barrow's Book of Nothing (2001) and other recent popular studies in mathematical thought. First printing of 50,000
"A wide-ranging exploration of the phenomenon of symmetry....There's math, yes, but there are also tales of love, violence, history and the whole, in this case, turns out to be greater than the sum of those parts." Mary Carmichael, Newsweek
"Fascinating. . . . [Livio] writes passionately about the role of symmetry in human perception and the arts." The Economist
"A lively and fascinating read for a broad audience." Nature
Product Details
BN ID: | 2940172640278 |
---|---|
Publisher: | Brilliance Audio |
Publication date: | 11/06/2018 |
Edition description: | Unabridged |
Read an Excerpt
The Equation that Couldn't Be Solved
How Mathematical Genius Discovered the Language of SymmetryBy Mario Livio
Simon & Schuster
Copyright © 2005 Mario LivioAll right reserved.
ISBN: 0743258207
Chapter One: Symmetry
An inkblot on a piece of paper is not particularly attractive to the eye, but if you fold the paper before the ink dries, you may get something that looks like figure 1 that is much more intriguing. In fact, the interpretation of similar inkblots forms the basis for the famous Rorschach test developed in the 1920s by the Swiss psychiatrist Hermann Rorschach. The declared purpose of the test is to somehow elicit the hidden fears, wild fantasies, and deeper thoughts of the viewers interpreting the ambiguous shapes. The actual value of the test as an "x-ray of the mind" is vehemently debated in psychological circles. As Emory University psychologist Scott Lilienfeld once put it, "Whose mind, that of the client or the examiner?" Nevertheless, there is no denial of the fact that images such as that in figure 1 convey some sort of attractive and fascinating impression. Why?
Is it because the human body, most animals, and so many human artifacts possess a similar bilateral symmetry? And why do all those zoological features and creations of the human imagination exhibit such a symmetry in the first place?
Most people perceive harmonious compositions such as Botticelli's Birth of Venus as symmetrical. Art historian Ernst H. Gombrich even notes that the "liberties which Botticelli took with nature in order to achieve a graceful outline add to the beauty and harmony of the design." Yet mathematicians will tell you that the arrangements of colors and forms in that painting are not symmetric at all in the mathematical sense. Conversely, most nonmathematical viewers do not perceive the pattern in figure 3 as symmetrical, even though it actually is symmetrical according to the formal mathematical definition. So what is symmetry really? What role, if any, does it play in perception? How is it related to our aesthetic sensibility? In the scientific realm, why has symmetry become such a pivotal concept in our ideas about the cosmos around us and in the fundamental theories attempting to explain it? Since symmetry spans such a wide range of disciplines, what "language" and what "grammar" do we use to describe and characterize symmetries and their attributes, and how was that universal language invented? On a lighter note, can symmetry provide an answer to the all-important question posed in the title of one of the songs of rock star Rod Stewart -- "Do Ya Think I'm Sexy?"
I will try to provide at least partial answers to all of these questions and many more. Along the way, I hope that the story as a whole will depict both the humanistic side of mathematics and, even more importantly, the human side of mathematicians. As we shall see, symmetry is the paramount tool for bridging the gap between science and art, between psychology and mathematics. It permeates objects and concepts ranging from Persian carpets to the molecules of life, from the Sistine Chapel to the sought-after "Theory of Everything." Yet group theory, the mathematical language that describes the essence of symmetries and explores their properties, did not emerge from the study of symmetries at all. Rather, this astonishingly unifying idea of modern thought emanated from a most unlikely source -- an equation that couldn't be solved. The dramatic and tortuous history of this equation is an essential part of this intellectual saga. At the same time, this tale will shed light on the loneliness of genius and on the tenacity of the human intellect in the face of seemingly insurmountable challenges. I have put a tremendous effort into trying to solve the two-centuries-old mystery of the death of the protagonist of this story -- the brilliant mathematician Evariste Galois. I believe that I have come closer to the truth than was ever possible before.
The witty playwright George Bernard Shaw once said, "The reasonable man adapts himself to the world; the unreasonable one persists in trying to adapt the world to himself. Therefore all progress depends on the unreasonable man." In this book we shall encounter many unreasonable men and women. The creative process, by its very nature, seeks uncharted intellectual and emotional terrain. Brief forays into mathematical abstraction will offer a peek into the very nature of creativity. I begin with a concise exploration of the wonderland of symmetries.
The word symmetry has ancient roots, coming from the Greek sym and metria, which translate into "the same measure." When the Greeks labeled a work of art or an architectural design symmetric, they meant that one could identify some small piece of the work, such that the dimensions of all the other parts contained that piece a precise number of times (the parts were "commensurable"). This early definition corresponds more to our modern notion of proportion than to symmetry. Nevertheless, the great philosophers Plato (428/427-348/347 BC) and Aristotle (384-322 BC) were quick to associate symmetry with beauty. In Aristotle's words, "The chief forms of beauty are orderly arrangement [in Greek taxis], proportion [symmetria], and definiteness [horismenon], which are revealed in particular by mathematics." Following in the Greeks' footsteps, the identification of symmetry with "due proportion" was subsequently propagated by the influential Roman architect Vitruvius (ca. 70-25 BC), and it persisted all the way through the Renaissance. In his De Architectura Libri Decem (Ten Books on Architecture), literally the architectural bible in Europe for centuries, Vitruvius writes:
The design of a temple depends on symmetry, the principles of which must be carefully observed by the architect. They are due to proportion. Proportion is a correspondence among the measures of the members of an entire work, and of the whole to a certain part selected as standard. From this result the principles of symmetry.
The modern meaning of symmetry (first introduced in the late eighteenth century) in the precise mathematical sense is really "immunity to a possible change." Or, as mathematician Hermann Weyl (1885-1955) once put it, "A thing is symmetrical if there is something you can do to it so that after you have finished doing it it looks the same as before." Examine for example the verses
Is it odd how asymmetricalIs "symmetry"?
"Symmetry" is asymmetrical.
How odd it is.
This stanza remains unchanged if read word by word from the end to the beginning -- it is symmetrical with respect to backward reading. If you envision the words as being arranged like beads along a string, you could regard this reverse reading as a sort of (not literal) mirror reflection of the stanza. This stanza does not change when mirror-reflected in the above sense -- it is symmetrical with respect to such mirror reflection. Alternatively, if you prefer to think in terms of reading the poem out loud, then the backward reading corresponds to a time reversal, somewhat like rewinding a videotape (again, not literally, because the individual sounds are not reversed). Phrases with this property are called palindromes.
The invention of palindromes is generally attributed to Sotades the Obscene of Maronea, who lived in the third century BC in Greek-dominated Egypt. Palindromes have been extremely popular with many word-play wizards such as the Englishman J. A. Lindon, and with the superb recreational-mathematics author Martin Gardner. One of Lindon's amusing word-unit palindromes reads: "Girl, bathing on Bikini, eyeing boy, finds boy eyeing bikini on bathing girl." Other palindromes are symmetric with respect to back-to-front reading letter by letter -- "Able was I ere I saw Elba" (attributed jokingly to Napoleon), or the title of a famous NOVA program: "A Man, a Plan, a Canal, Panama."
Surprisingly, palindromes appear not just in witty word games but also in the structure of the male-defining Y chromosome. The Y's full genome sequencing was completed only in 2003. This was the crowning achievement of a heroic effort, and it revealed that the powers of preservation of this sex chromosome have been grossly underestimated. Other human chromosome pairs fight damaging mutations by swapping genes. Because the Y lacks a partner, genome biologists had previously estimated that its genetic cargo was about to dwindle away in perhaps as little as five million years. To their amazement, however, the researchers on the sequencing team discovered that the chromosome fights withering with palindromes. About six million of its fifty million DNA letters form palindromic sequences--sequences that read the same forward and backward on the two strands of the double helix. These copies not only provide backups in case of bad mutations, but also allow the chromosome, to some extent, to have sex with itself -- arms can swap position and genes are shuffled. As team leader David Page of MIT has put it, "The Y chromosome is a hall of mirrors."
Of course, the most familiar example of mirror-reflection symmetry is that of the bilateral symmetry that characterizes the animal kingdom. From butterflies to whales, and from birds to humans, if you reflect the left half in a mirror you obtain something that is almost identical to the right half. I will, for the moment, ignore the small if tantalizing external differences that do exist, and also the fact that neither the internal anatomy nor the functions of the brain possess bilateral symmetry.
To many, the word symmetry is actually assumed to mean bilateral symmetry. Even in Webster's Third New International Dictionary, one of the definitions reads: "Correspondence in size, shape, and relative position of parts that are on opposite sides of a dividing line or median plane." The precise mathematical description of reflection symmetry uses the same concepts. Take a drawing of a bilaterally symmetric butterfly and mark a straight line down the middle of the figure. If you flip the drawing over keeping the central line in place, perfect overlapping will occur. The butterfly remains unchanged -- invariant -- under reflection about its central line.
Bilateral symmetry is so prevalent in animals that it can hardly be due to chance. In fact, if you think of animals as vast collections of trillions and trillions of molecules, there are infinitely more ways to construct asymmetrical configurations out of these building blocks than symmetrical ones. The pieces of a broken vase can lie in a pile in many different assortments, but there is only one arrangement in which they all fit together to reproduce the intact (and usually bilaterally symmetric) vase. Yet the fossil record from the Ediacara Hills of Australia shows that soft-bodied organisms (Spriggina) that date back to the Vendian period (650 to 543 million years ago) already exhibited bilateral symmetry.
Since life forms on Earth were shaped by eons of evolution and natural selection, these processes must have somehow preferred bilateral or mirror symmetry. Of all the different guises animals could have taken, bilaterally symmetrical ones had superiority. There is no escape from the conclusion that this symmetry was a likely outcome of biological growth. Can we understand the cause for this particular predilection? We can at least try to find some of its engineering roots in the laws of mechanics. One key point here is the fact that all directions on the surface of the Earth were not created equal. A clear distinction between up and down (dorsal and ventral in animals, in the biological jargon) is introduced by the Earth's gravity. In most cases what goes up must come down, but not the other way around. Another distinction, between front and back, is a result of animal locomotion.
Any animal moving relatively rapidly, be it in the sea, on land, or in the air, has a clear advantage if its front is different from its rear end. Having all the sensory organs, the major detectors of light, sound, smell, and taste, in the front clearly helps the animal in deciding where to go and how to best get there. A frontal "radar" also provides an early warning against potential dangers. Having the mouth in the front can make all the difference between reaching lunch first or not. At the same time, the actual mechanics of movement (especially on land and in the air) under the influence of the Earth's gravitational force have generated a clear difference between bottom and top. Once life emerged from the sea and onto the land, some sort of mechanical devices--legs--had to develop to carry the animal around. No such appendages were needed at the top, so the difference between top and bottom became even more pronounced. The aerodynamics of flying (still under Earth's gravity) coupled with the requirements for a landing gear plus some means of movement on the ground combined to introduce top-bottom differences in birds.
Here, however, comes an important realization: There is nothing major in the sea, on the ground, or in the air, to distinguish between left and right. The hawk looking to the right sees just about the same environment it sees to the left. The same is not true about up and down -- up is where the hawk flies even higher into the sky, while down is where it lands and builds its nest. Political puns aside, there really is no big difference between left and right on Earth, because there are no strong horizontal forces. To be sure, the Earth's rotation around its axis and the Earth's magnetic field (the fact that Earth acts on its surroundings like a bar magnet) do introduce an asymmetry. However, these effects are not nearly as significant at the macroscopic level as those of gravity and rapid animal motion.
The description so far explains why bilateral symmetry of living organisms makes sense mechanically. Bilateral symmetry is also economical -- you get two organs for the price of one. How this symmetry or lack thereof emerged from evolutionary biology (the genes) or even more fundamentally from the laws of physics is a more difficult question, to parts of which I shall return in chapters 7 and 8. Here let me note that many multicellular animals have an early embryonic body that lacks bilateral symmetry. The driving force behind the modification of the "original plan" as the embryo grows may indeed be mobility.
Not all animate nature lives in the fast lane. Life forms that are anchored in one place and are unable to move voluntarily, such as plants and sessile animals, do have very different tops and bottoms, but no distinguishable front and back or left and right. They have symmetry similar to that of a cone -- they produce symmetrical reflections in any mirror passing through their central, vertical axis. Some animals that move very slowly, such as jellyfish, have a similar symmetry.
Obviously, once bilateral symmetry had developed in living creatures, there was every reason to keep it intact. Any loss of an ear or an eye would make an animal much more vulnerable to a predator sneaking up on it unnoticed.
One may always wonder whether the particular standard configuration nature has endowed humans with is the optimal one. The Roman god Janus, for example, was the god of the gates and of new beginnings, including the first month (January) of the year. Accordingly, he is always depicted in art with two faces, one in the front facing forward (symbolically toward the coming year) and one at the back of the head (toward the year that has passed). Such an arrangement in humans, while useful for some purposes, would have left no space for the parts of the brain that are responsible for the nonsensory systems. In his wonderful book The New Ambidextrous Universe, Martin Gardner tells the story of a Chicago entertainer who had a routine discussing the advantages of having various sensory organs at unusual spots on the body. Ears under the armpits, for instance, would be kept warm in the cold Chicago winters. Clearly, other shortcomings would be associated with such a configuration. The hearing of armpit ears would be seriously impaired unless you kept your arms raised all the time.
Science-fiction movies invariably feature aliens that are bilaterally symmetric. If extraterrestrial intelligent creatures that have evolved biologically exist, how likely are they to possess reflection symmetry? Quite likely. Given the universality of the laws of physics, and in particular the laws of gravity and motion, life forms on planets outside the solar system face some of the same environmental challenges that life on Earth does. The gravitational force still holds everything on the surface of the planet and creates a significant discrimination between up and down. Locomotion similarly separates the front end from the rear. E.T. is or was most likely ambidextrous. This does not mean, however, that any delegation of visiting aliens would look anything like us. Any civilization sufficiently evolved to engage in interstellar travel has likely long passed the merger of an intelligent species with its far superior computational-technology-based creatures. A computer-based super-intelligence is most likely to be microscopic in size.
Some of the capital letters in the alphabet are among the numerous human-created objects that are symmetric with respect to mirror reflections. If you hold a sheet of paper with the letters A, H, I, M, O, T, U, V, W, X, Y up to a mirror, the letters look the same. Words (or even entire phrases) constructed from these letters and printed vertically, such as the not-too-deep instruction remain unchanged when mirror reflected. The Swedish pop-music group ABA, whose music inspired the successful musical Mamma Mia, introduced a trick into the spelling of its name that makes it mirror symmetric (MAMMA MIA written vertically is also mirror symmetric). A few letters, such as B, C, D, E, H, I, K, O, X, are symmetric with respect to reflection in a mirror that bisects them horizontally. Words composed of these letters, such as COOKBOOK, BOX, CODEX, or the familiar symbols for hugs and kisses, XOXO, remain unchanged when held upside down to a mirror.
The importance of mirror-reflection symmetry to our perception and aesthetic appreciation, to the mathematical theory of symmetries, to the laws of physics, and to science in general, cannot be overemphasized, and I will return to it several times. Other symmetries do exist, however, and they are equally relevant.
The title of this section is taken from "The Snowstorm" by the American poet and essayist Ralph Waldo Emerson (1803-82). It expresses the bewilderment one feels upon discerning the spectacular shapes of snowflakes. While the common phrase "no two snowflakes are alike" is actually not true at the naked-eye level, snowflakes that have formed in different environments are indeed different. The famous astronomer Johannes Kepler (1571-1630), who discovered the laws of planetary motion, was so impressed with the marvels of snowflakes that he devoted an entire treatise, The Six-Cornered Snowflake, to the attempt to explain the symmetry of snowflakes. In addition to mirror-reflection symmetry, snowflakes possess rotational symmetry -- you can rotate them by certain angles around an axis perpendicular to their plane (passing through the center) and they remain the same. Due to the properties and shape of water molecules, snowflakes have typically six (almost) identical corners. Consequently, the smallest rotation angle (other than no rotation at all) that leaves the shape unchanged is one in which each corner is displaced by one "step": 360 4 6 5 60 degrees. The other angles that lead to an indistinguishable final figure are simple multiples of this angle: 120, 180, 240, 300, 360 degrees (the last one returns the snowflake to its original position and is equivalent to no rotation at all). Snowflakes therefore have sixfold rotational symmetry. By comparison, starfish have fivefold rotational symmetry; they can be rotated by 72, 144, 216, 288, and 360 degrees with no discernable difference. Many flowers, such as the chrysanthemum, the English daisy, and the tickseed (coreopsis), display an approximate rotational symmetry. They look essentially the same when rotated by any angle. Symmetry, when combined with rich colors and intoxicating smells, is an underlying property that gives flowers their universal aesthetic appeal. Perhaps no one has expressed better the associative relationship between flowers and works of art than the painter James McNeill Whistler (1834-1903):
The masterpiece should appear as the flower to the painter--perfect in its bud as in its bloom -- with no reason to explain its presence -- no mission to fulfill -- a joy to the artist, a delusion to the philanthropist -- a puzzle to the botanist -- an accident of sentiment and alliteration to the literary man.
What is it in a symmetric pattern that provokes such an emotional response? And is this truly the same excitement that is stimulated by works of art? Note that even if the answer to the latter question is an unequivocal yes, this does not necessarily bring us any closer to answering the first question. The answer to the question, What is it in works of art that provokes an emotional response? is far from clear. Indeed, what quality is shared by such different masterpieces as Jan Vermeer's Girl with a Pearl Earring, Pablo Picasso's Guernica, and Andy Warhol's Marilyn Diptych? Clive Bell (1881-1964), an art critic and member of the Bloomsbury group (which, by the way, included novelist Virginia Woolf), suggested that the one quality common to all true works of art was what he called "significant form." By this he meant a particular combination of lines, colors, forms, and relations of forms that stirs our emotions. This is not to say that all works of art evoke the same emotion. Quite the contrary: every work of art may evoke an entirely different emotion. The commonality is in the fact that all works of art do evoke some emotion. If we were to accept this aesthetic hypothesis, then symmetry may simply represent one of the components of this (rather vaguely defined) significant form. In this case, our reaction to symmetric patterns may not be too different (even if less intense perhaps) from our broader aesthetic sensibility. Not all agree with such an assertion. Aesthetics theorist Harold Osborne had this to say about the human response to symmetry of individual elements or objects, such as snowflakes: "They can arouse interest, curiosity and admiration. But visual interest in them is short-lived and superficial: In contrast to the impact of an artistic masterpiece, perceptual attention soon wanders, never goes deep. There is no enhancement of perception." Actually, as I will show in the next chapter and in chapter 8, symmetry has much to do with perception. For the moment, however, let me concentrate on the purely aesthetic "value" of symmetry.
Dartmouth College psychologists Peter G. Szilagyi and John C. Baird conducted a fascinating experiment in 1977 that was intended to explore the quantitative relationship between the amount of symmetry in designs and aesthetic preference. Twenty undergraduate students (the most common subjects of experimental psychology) were asked to perform three simple tasks. In the first, they were invited to arrange eight squares with a black dot at their centers inside a row of eighteen cells, each of a size equal to that of the squares. The instructions to the subjects were to arrange the pieces in a manner that they found "visually pleasing." Each piece had to cover one cell entirely, and all the squares had to be used. The second and third tasks were similar in nature. In the second, eleven pieces had to be arranged in a 5 3 5 grid. In the third, twelve cubes had to be fitted into holes in a three-dimensional transparent structure consisting of three horizontal planes, each containing nine square holes. The results showed an unambiguous aesthetic preference for symmetrical designs. For instance, 65 percent of the subjects created perfect mirror-reflection-symmetric patterns in the first task. In fact, symmetry was the primary component in the designs of most subjects (in one, two, and three dimensions), with perfect symmetry being the most favored condition.
The association between symmetry and artistic taste emerged not just in experiments, but also in a more speculative theory of aesthetics developed by the famous Harvard mathematician George David Birkhoff (1884-1944). Birkhoff is best known for proving in 1913 a famous geometric conjecture formulated by the French mathematician Henri Poincare, and for his ergodic theorem (published in 1931-32) -- a contribution of paramount significance for the theory of gases and for probability theory. During his undergraduate days, Birkhoff started to be intrigued by the structure of music, and around 1924 he expanded his interests to aesthetics in general. In 1928, he spent half a year traveling extensively in Europe and the Far East in an attempt to absorb as much art, music, and poetry as he could. His efforts to develop a mathematical theory of aesthetic value culminated in the publication of Aesthetic Measure in 1933. Birkhoff specifically discusses the intuitive feeling of value evoked by works of art, which is "clearly separable from sensuous, emotional, moral, or intellectual feeling." He separates the aesthetic experience into three phases: (1) the effort of attention necessary for perception; (2) the realization that the object is distinguished by a certain order; (3) the appreciation of value that rewards the mental effort. Birkhoff further assigns quantitative measures to the three stages. The preliminary effort, he suggests, increases in proportion to the complexity of the work (denoted by C). Symmetries play a key role in the order (denoted by O) characterizing the object. Finally, the feeling of value is what Birkhoff calls the "aesthetic measure" (denoted by M) of the work of art.
The essence of Birkhoff's theory can be summarized as follows. Within each class of aesthetic objects, such as ornaments, vases, pieces of music, or poetry, one can define an order O and a complexity C. The aesthetic measure of any object in the class can then be calculated simply by dividing O by C. In other words, Birkhoff proposed a formula for the feeling of aesthetic value: M 5 O 4 C. The meaning of this formula is: For a given degree of complexity, the aesthetic measure is higher the more order the object possesses. Alternatively, if the amount of order is specified, the aesthetic measure is higher the less complex the object. Since for most practical purposes, the order is determined primarily by the symmetries of the object, Birkhoff's theory heralds symmetry as a crucial aesthetic element.
Birkhoff was the first to admit that the precise definitions of his elements O, C, and M were tricky. Nevertheless, he made a valiant attempt to provide detailed prescriptions for the calculation of these measures for a variety of art forms. In particular, he started with simple geometrical shapes, continued with ornaments and Chinese vases, proceeded to harmony in the diatonic musical scale, and concluded with the poetry of Tennyson, Shakespeare, and Amy Lowell.
No one, especially not Birkhoff himself, would claim that the intricacies of aesthetic pleasure could be reduced entirely to a mere formula. However, in Birkhoff's words, "In the inevitable analytic accompaniment of the creative process, the theory of aesthetic measure is capable of performing a double service: it gives a simple, unified account of the aesthetic experience, and it provides means for the systematic analysis of typical aesthetic fields."
Returning now from this brief detour into the land of aesthetics to the specific case of rotational symmetry, we note that one of the simplest rotationally symmetric figures in the plane is a circle. If you rotate it around its center through, say, 37 degrees, it remains unchanged. In fact you can rotate it through any angle around a perpendicular axis through its center and you will not notice any difference. The circle therefore has an infinite number of rotational symmetries. These are not the only symmetries the circle possesses. Reflections in all the axes that cut along a diameter (figure 8b) also leave the circle unchanged.
The same system can, therefore, have multiple symmetries, or be symmetric under a variety of symmetry transformations. Rotating a perfect sphere about its center, using an axis running in any direction, leaves it looking precisely the same. Or examine, for instance, the equilateral (all sides the same) triangle. We are allowed neither to change the shape or size of this triangle, nor to move it about. What transformations could we apply to it to leave it unchanged? We could rotate it by 120, 240, and 360 degrees around an axis perpendicular to the plane of the figure and passing through point O. These transformations do interchange the locations of the vertices, but if you turn your back while somebody is performing these rotations you won't notice anything different. Note that a rotation by 360 degrees is equivalent to doing nothing at all, or rotating by zero degrees. This is known as the identity transformation. Why bother to define such a transformation at all? As we shall see later in the book, the identity transformation plays a similar role to that of the number zero in the arithmetic operation of addition or the number one in multiplication -- when you add zero to a number or multiply a number by one, the number remains unchanged. We can also mirror-reflect the triangle about the three dashed lines in figure 9c. There are, therefore, precisely six symmetry transformations -- three rotations and three reflections -- associated with the equilateral triangle.
What about combinations of some of these transformations, such as a reflection followed by a rotation? Don't they add to the number of symmetries of the triangle? I shall return to this question in the context of the language of symmetries. For the moment, however, another important symmetry awaits exposition.
One of the most familiar of all symmetric patterns is that of a repeating, recurring motif. From friezes of classical temples and pillars of palaces to carpets and even birdsong, the symmetry of repeating patterns has always produced a very comforting familiarity and a reassuring effect.
The symmetry transformation in this case is called translation, meaning a displacement or shift by a certain distance along a certain line. The pattern is called symmetric if it can be displaced in various directions without looking any different. In other words, regular designs in which the same theme repeats itself at fixed intervals possess translational symmetry. Ornaments that are symmetric under translation can be traced all the way back to 17,000 BC (the Paleolithic era). A mammoth-ivory bracelet found in the Ukraine is marked with a repeating zigzag pattern. Other translation-symmetric designs are found in a variety of art forms ranging from medieval Islamic tiling in the Alhambra palace in Granada, Spain, through Renaissance typography, to the drawings of the fantastic Dutch graphic artist M. C. Escher (1898-1972). Nature also provides examples of translation-symmetric creatures, such as the centipedes, in which identical body segments may repeat as many as 170 times.
The Victorian artist, poet, and printer William Morris (1834-96) was a prolific producer of decorative art. Much of his work is literally the embodiment of translational symmetry. Early in life, Morris became fascinated by medieval architecture, and at age twenty-seven he started a firm of decorators that later became famous as Morris and Company. In a strong reaction to the increasing industrialism in nineteenth-century England, Morris looked for ways to revive artistic craftsmanship and to revitalize the splendor of the decorative arts of the Middle Ages. Morris and Company, and later the Kelmscott Press founded by Morris in 1890, designed spectacular tiles, tableware, textiles, and illustrated manuscripts in medieval design. But it was in wallpaper design where Morris first achieved his incredible mastery of translation-symmetric repeating patterns. A couple of his sumptuous themes are shown in figure 11. While Morris's designs may not have been any more innovative than those of some of his contemporaries, such as Christopher Dresser or A. W. N. Pugin, his influence and legacy have been enormous. Morris himself was interested in promoting arts and crafts and not in the mathematics of symmetry. In The Beauty of Life he summarized his socio-aesthetic philosophy this way:
You may hang your walls with tapestry instead of whitewash or paper; or you may cover them with mosaic; or have them frescoed by a great painter: all this is not luxury, if it be done for beauty's sake, and not for show: it does not break our golden rule: Have nothing in your houses which you do not know to be useful or believe to be beautiful.
An interesting question is whether symmetry with respect to translation, and indeed reflection and rotation too, is limited to the visual arts, or may be exhibited by other artistic forms, such as pieces of music. Evidently, if we refer to the sounds, rather than to the layout of the written musical score, we would have to define symmetry operations in terms other than purely geometrical, just as we did in the case of the palindromes. Once we do that, however, the answer to the question, Can we find translation-symmetric music? is a resounding yes. As Russian crystal physicist G. V. Wulff wrote in 1908: "The spirit of music is rhythm. It consists of the regular, periodic repetition of parts of the musical composition...the regular repetition of identical parts in the whole constitutes the essence of symmetry." Indeed, the recurring themes that are so common in musical composition are the temporal equivalents of Morris's designs and symmetry under translation. Even more generally, compositions are often based on a fundamental motif introduced at the beginning and then undergoing various metamorphoses.
Simple examples of symmetry under translation in music include the opening measures in Mozart's famous Symphony no. 40 in G Minor, as well as the entire structure of some common musical forms. In the former example you can see the translational symmetry not only within each line of the score (where the short declining gestures are marked), but also between the first line and the second (denoted by a and b). In terms of overall design, if we use the symbols A, B, and C to describe entire sections of a movement, then the pattern for a rondo as a whole, for instance, can be expressed as ABACA or ABACABA, where the translational symmetry is apparent. Mozart's association with objects of mathematics should come as no surprise. His sister, Nannerl, recalled that he once covered the walls of the staircase and of all the rooms in their house with numbers, and when no space remained, he moved on to the walls of a neighboring house. Even the margins of Mozart's manuscript for the Fantasia and Fugue in C Major contain calculations of the probability to win the lottery. No wonder then that British musicologist and composer Donald Tovey identified the "beautiful and symmetrical proportions" of Mozart's compositions as one of the key reasons for their popularity.
Another great composer known for his obsession with numbers, mental games, and their use in complex musical form was Johann Sebastian Bach (1685-1750). Both reflection and translation feature frequently in Bach's music on many levels. An example encompassing reflection by a horizontal "mirror" is the opening of Bach's Two-Part Invention no. 6 in E Major. Imagine a mirror in the space between the two score lines. The ascending trend marked by line a is reflected (half a bit later) by the descending trend b, and the entire gesture is reflected and repeated again slightly later (starting at d). Another example is provided by the entire large-scale structure of one of Bach's most notable works, the famous Musical Offering. The composition consists of these musical forms:
Ricercar 5 Canons Trio Sonata 5 CanonsRicercar
It exhibits reflection symmetry (obviously not sound by sound).
Ricercar (from ricercare--"to research, or seek out") was an old term used loosely for any type of prelude, usually in fugal style. The great humanitarian, physician, and philosopher Albert Schweitzer (1875-1965) was also a great Bach enthusiast. In his book J. S. Bach he notes: "The word [ricercar] signifies a piece of music in which we have to seek something -- namely a theme." The Musical Offering also contains ten canons, which, by construction, involve the operation of translation. In any canon (the word means "rule"), one melodic strand determines the rule (in terms of melodic line or rhythm) for the second or more voices. The second voice follows at some fixed interval of time -- a temporal translation. A simple, familiar example is
Row row row your boatGently down the stream
Merrily merrily merrily merrily
Life is but a dream,
where the second voice starts when the first reaches the word "gently."
The story surrounding the Musical Offering is in itself truly fascinating. Three years before his death, Bach was on his way to Berlin to visit his daughter-in-law Johanna Maria Dannemann (wife of the composer Carl Philipp Emanuel Bach), who was at the time expecting a child. Exhausted from the long journey, the aged composer made a stop at Potsdam, then the seat of King Frederick the Great of Prussia, who also employed Carl Philipp Emanuel. The news of Bach's arrival at the royal palace prompted the king to cancel a planned evening concert featuring himself playing on the flute in favor of an impromptu series of recitals by Bach on seven new fortepianos. Gottfried Silbermann, the master organ builder of the German baroque, constructed these instruments. Following a virtuoso performance in seven different rooms of the palace, Bach offered to his delighted audience to improvise a fugue on a theme His Royal Highness would suggest. Upon returning home, Bach developed the Musical Offering from that improvised fugue. He added to it a set of magnificently complex canons and a trio sonata and elaborated upon the other contrapuntal movements. The sonata featured a flute (King Frederick's instrument), a violin, and continuo (keyboard and cello). For the title of the Offering, the ever word-playful Bach chose Regis iussu cantio et reliqua canonica arte resoluta (Upon the King's Demand the Theme and Additions Resolved in Canonic Style), which forms the acronym RICERCAR.
There are even more symmetries in the Musical Offering. In Canon I (the Crab Canon), each violin plays the other's part backward, resulting in reflection symmetry (of the score) in a vertical mirror. Finally, canons in general were considered at the time to be some sort of symmetry puzzles. The composer provided the theme, but it was the musicians' task to figure out what type of symmetry operation he had in mind for the theme to be performed. In the case of the Musical Offering, Bach accompanied the last two canons before the trio sonata with the inscription "Quaerendo inventis," meaning "Seek, and ye shall find." As we shall see in chapter 7, this is not very different conceptually from the puzzle posed to us by the universe -- it lies in all its glory open to inspection--for us to find the underlying patterns and symmetries. Even the uncertainties and ambiguities involved in the attempts to uncover the "theory of everything" may have an analogy in Bach's intellectual challenge. You see, one of the canons in the Musical Offering has three possible solutions.
Translation and reflection can be combined into one symmetry operation known as glide reflection. The footprints generated by an alternating left-right-left-right walk exhibit glide-reflection symmetry. The operation consists simply of a translation (the glide), followed by a reflection in a line parallel to the direction of the displacement (the dashed line in the figure). Equivalently, you could look at glide reflection as a mirror reflection followed by a translation parallel to the mirror. Glide-reflection symmetry is common in classical friezes, and also in the ceramics of native Americans in New Mexico. Whereas patterns that are translation symmetric tend to convey an impression of motion in one direction, glide-reflection-symmetric designs create a snakelike visual sensation. Real snakes achieve these patterns by alternately contracting and relaxing muscle groups on both sides of their body -- when they contract a group on the right, the corresponding group on the left is relaxed, and vice versa.
We have by now encountered all the rigid transformations that result in symmetries in two dimensions. The word rigid simply means that after the transformation every two points end up the same distance apart as they were to begin with -- we cannot shrink figures, inflate them, or deform them.
In three-dimensional space, in addition to the symmetry under translation, rotation, reflection, and glide reflection, we can find yet another symmetry known as screw symmetry. This is the type of symmetry of a corkscrew, where rotation about some axis is combined with translation along that axis. Some stems of plants, where the leaves appear at regular intervals after completing the same fraction of a full circle around the stem, possess this symmetry. Are these all the symmetries that exist? Definitely not.
All Are Equal, But...
The arts and sciences are chock-full of fascinating examples of symmetry under the operations of translation, rotation, reflection, and glide reflection, and we shall return to some of those in later chapters.
Permutations feature in such diverse circumstances as the changing of partners in Scottish folk dancing and shuffled decks of cards. The main concern of the operation of permutations is not so much with which object lies where, as with which object takes the place of which. For example, in the permutation: 1 2 3 4 -> 4 1 3 2, the number 1 was replaced by 4, 2 was replaced by 1, 3 stayed put, and 4 was replaced by 2. This is usually denoted by
4132'
where each number in the upper row is replaced by the number directly underneath. The same permutation operation could have been written as
3142
because precisely the same replacements took place, and the order in which the numbers are written is not important. You may wonder how can a system be symmetric (i.e., not change) under permutations? Evidently, if you have ten books on a shelf and they are all different, any permutation that is not the identity (leaving the books untouched) will change the order. However, if you have three copies of the same book, for example, clearly some permutations will leave the order unchanged. The English essayist and critic Charles Lamb (1775-1834), known for his self-revealing observations of life, had a rather strong opinion on some such book "rearrangements." He writes, "The human species, according to the best theory I can form of it, is composed of two distinct races, the men who borrow, and the men who lend....Your borrowers of books -- those mutilators of collections, spoilers of the symmetry of shelves, and creators of odd volumes."
Symmetry under permutation can appear in more abstract circumstances. Examine the contents of the phrase "Rachel is David's cousin." The meaning will remain unchanged if we interchange David with Rachel. The same is not true for the phrase "Rachel is David's daughter." Similarly, the equality between two quantities, a 5 b, is symmetric under the transposition of a and b, since b 5 a is the same relation. While this may seem trivial, the relation "greater than" (commonly denoted by the symbol $) does not have this property. The relation a $ b means "a is greater than b." Permuting the letters results in b $ a, "b is greater than a," and the two relations are mutually exclusive.
Various mathematical formulae can also be symmetric under permutations. The value of the expression ab 1 bc 1 ca (where ab means "a times b" and so on) remains unchanged under any permutation of the letters a, b, c. As I shall discuss in more detail later, there are precisely six possible permutations of three letters, including one (the first below) that is the identity, mapping each letter into itself:
abc acb bca cab cba bac
You can easily check that the above expression is unaltered by these permutations. For instance, the third permutation changes a into b, b into c, and c into a. The entire formula therefore changes to read: bc 1 ca 1 ab. However, since in whichever order we either multiply numbers or add them up the result is always the same, the new expression is equal to the original one.
People playing roulette in a casino provide an interesting case of symmetry under permutations. The roulette is composed of a rotating wheel in which eighteen numbered red slots, eighteen black slots, and two green slots commonly labeled 0 and 00 are marked. A white ball is dropped onto the spinning wheel, and after rolling rapidly around the rim a few times, it bounces around and eventually lands and comes to rest in one of the slots. When the wheel is mechanically perfect, the game of roulette is absolutely symmetric under any permutation of the players. Everybody has precisely the same chance to win or lose irrespective of whether they are casino rats or novices, experts in probability theory or village idiots. The expectation to win (rather to lose, about 5.3 cents for every dollar bet, on the average) does not depend on the amount of money being risked or on the player's strategy. While no mechanical wheel can be truly perfect, centuries of profits for casinos prove that, whatever small deviations may exist, they do not lead to a significant violation of the symmetry under permutations.
Not all gambling activities are symmetric under permutations of the players. Blackjack is a card game in which each gambler at the table plays against the dealer. Each number card has its face value, with all the picture cards having the value of ten, and the ace offering the option of being counted as one or eleven. The objective is to get the sum of the values of the dealt cards to be closer to twenty-one than the dealer's hand without exceeding twenty-one. What makes blackjack asymmetric with respect to permutations of the players is precisely the fact that strategy is important. In the 1960s casinos discovered the hard way the extent to which strategy counts. Mathematician Edward O. Thorp uncovered a flaw in the way casinos were calculating probabilities when the deck of cards was dwindling down. He used this information to develop an extremely profitable method of play. In case you wonder, the casinos have since taken corrective action. Nonetheless, it remains true that strategy does make a difference in blackjack. Indeed, six MIT students who communicated with card-count code words made millions in Las Vegas in the 1990s.
Permutation symmetry and some of its scientific close cousins have far-reaching consequences in the physics of the subatomic world, and we shall return to some of those in chapter 7. Here I will only mention briefly one simple example that explains an otherwise perplexing fact about atoms of different elements -- they are all roughly the same size.
Atoms somewhat resemble miniature solar systems. The electrons in the atom are orbiting a central nucleus, just as the planets are revolving around the sun. The force that holds the electrons in their orbits, however, is electromagnetic, rather than gravitational. The nucleus contains protons that have positive electric charges (and neutrons, which are neutral), while the orbiting electrons (equal in number to the protons) are negatively charged. Opposite electric charges attract each other. Unlike planetary systems, which can have orbits of any size, atoms must obey the rules of the subatomic realm -- quantum mechanics. The highest probability of finding the electrons is along certain specific, "quantized" orbits, restricted to a particular series of discrete sizes. The permitted orbits are characterized mainly by their energy. Broadly speaking, the higher the energy associated with the orbit, the larger its size. The situation is somewhat analogous to a flight of stairs, with the nucleus representing the bottom and the higher energy levels corresponding to increasingly higher steps. Here, however, comes the puzzle. Physics, and indeed everyday life, teach us that systems are most stable in their lowest possible energy state (e.g., a ball rolling down the steps reaches stability at the bottom). This would mean that whether we are dealing with the hydrogen atom, which has only one electron, the oxygen atom, with eight electrons, or uranium, ninety-two electrons, all the electrons would be clustered in the smallest possible orbit. Because the more electron- and proton-rich atoms are, the stronger the electrical attraction between the nucleus and the electrons, we would expect that the oxygen atom would be smaller than the hydrogen atom, and the uranium atom much smaller still. Experiments show, however, that this is far from being the case. Rather, irrespective of the number of electrons, the atoms are found to be roughly the same size. Why?
The explanation was given by the famous physicist Wolfgang Pauli (1900-58). He proposed in 1925 a powerful law of nature (which won him the Nobel Prize in 1945), known as the Pauli exclusion principle. The law refers to some elementary particles of the same type, such as electrons. All the electrons in the universe are precisely identical in terms of their intrinsic properties -- there is no way to distinguish one from the other. In addition to their mass and electric charge, electrons have another fundamental property called spin. Spin could be thought of, for some purposes, as if the electron were a tiny ball spinning around its axis. Quantum mechanics -- the theory that describes atoms, light, and subatomic particles -- tells us that the electron spin can have only two states (loosely analogous to the ball spinning at a specific rate in one or the opposite direction). The Pauli exclusion principle asserts that no two electrons can be in precisely the same state; that is, having exactly the same orbit and direction of spin. How is this related to symmetry? To phrase the exclusion principle more accurately, we need to realize that quantum mechanics speaks in the language of probabilities. We can never determine precisely the location of an electron within the atom. Rather, we can only determine the different probabilities of finding it at various positions. The collection of all of these probabilities is known as the probability function. The probability function plays the role of a map, showing us where we are most likely to find the electron. Accordingly, Pauli also formulated his exclusion principle in terms of a property of the probability function describing the motion of electrons in the atom. He stated that the probability function is antisymmetrical with respect to interchanging any electron pair. Such a function is called antisymmetrical if transposing two electrons that move along the same orbit and have the same spin direction changes only the sign of the function (e.g., from plus to minus), but not its value. For instance, imagine that the letter a symbolizes the value of some property of the first electron, and the letter b the value of the same property for the second electron. A function that takes the value a 1 b is symmetrical under the exchange of the two electrons since a 1 b equals b 1 a. On the other hand, a function represented by a 2 b is antisymmetrical, since changing a to b and b to a changes a 2 b into b 2 a, and b 2 a is precisely the negative of a 2 b (e.g., 5 2 3 5 2; 3 2 5 5 22).
Pauli's statement is therefore the crux of the matter. On one hand, we know that if we interchange two identical electrons this should make no difference whatsoever, and the probability function should remain unchanged. On the other, the exclusion principle tells us that the probability function should change its sign (e.g., from positive to negative) under such a permutation. What kind of number is equal to the negative of itself? There is only one such number -- zero. Changing the sign in front of a zero does not change the value by one iota; minus zero equals plus zero. In other words, the probability of finding two electrons with the same spin moving along the same orbit is zero -- no such state exists.
Pauli's exclusion principle tells us that electrons with the same properties don't like to be bunched up in the same place. Consequently, no more than two electrons (one with each direction of spin) are allowed in any given orbit. Instead of all the electrons crowding the smallest (lowest energy) orbit, electrons are forced into successively higher-energy, larger-size orbits. The net result is that even though the sizes of all the quantized orbits are smaller in the heavier (more proton-rich) atoms, electrons have no choice but to occupy an increasing number of orbits. Amazingly, the behavior of the probability function under permutations of electrons provides the explanation why atoms are nearly equal in size.
Returning now to permutations in general, color transformation may be considered as near kin. For any pattern that has more than one color, such as a chessboard, the colors can be interchanged. Strictly speaking, actual patterns are usually not symmetrical under color transformation -- they do change. A few of the imaginative designs of M. C. Escher come just about as close as one can expect to being color symmetrical. Note that the image does not remain truly the same when black and white are transposed, and neither does a chessboard. However, the general visual impression remains the same.
Escher himself was never quite sure what had led him to his obsession with translation-symmetric, color-symmetric patterns. In his own words,
I often wondered at my own mania of making periodic drawings. Once I asked a friend of mine, a psychologist, about the reason of my being so fascinated by them, but his answer: that I must be driven by a primitive, prototypical instinct, does not explain anything. What can be the reason of my being alone in this field? Why does none of my fellow-artists seem to be as fascinated as I am by these interlocking shapes? Yet their rules are purely objective ones, which every artist could apply in his own personal way!
Escher's retrospective musings touch upon two important topics: the role of symmetry in the "primitive" process of perception, and the rules that underlie symmetry. The latter topic will be the subject of several later chapters. However, since all the information we obtain about the world comes through our senses, the question of symmetry as a potential factor in perception becomes of immediate relevance.
Copyright © 2005 by Mario Livio
Continues...
Excerpted from The Equation that Couldn't Be Solved by Mario Livio Copyright © 2005 by Mario Livio.
Excerpted by permission.
All rights reserved. No part of this excerpt may be reproduced or reprinted without permission in writing from the publisher.
Excerpts are provided by Dial-A-Book Inc. solely for the personal use of visitors to this web site.
Videos
