The Circuitry of the Human Spinal Cord: Its Role in Motor Control and Movement Disorders available in Hardcover
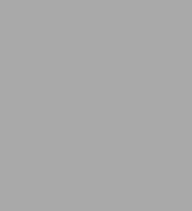
The Circuitry of the Human Spinal Cord: Its Role in Motor Control and Movement Disorders
- ISBN-10:
- 0521825814
- ISBN-13:
- 9780521825818
- Pub. Date:
- 06/08/2005
- Publisher:
- Cambridge University Press
- ISBN-10:
- 0521825814
- ISBN-13:
- 9780521825818
- Pub. Date:
- 06/08/2005
- Publisher:
- Cambridge University Press
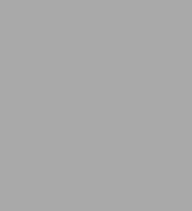
The Circuitry of the Human Spinal Cord: Its Role in Motor Control and Movement Disorders
Hardcover
Buy New
$415.00Buy Used
-
-
SHIP THIS ITEM
Temporarily Out of Stock Online
Please check back later for updated availability.
-
Overview
Product Details
ISBN-13: | 9780521825818 |
---|---|
Publisher: | Cambridge University Press |
Publication date: | 06/08/2005 |
Edition description: | New Edition |
Pages: | 664 |
Product dimensions: | 7.68(w) x 9.96(h) x 1.57(d) |
About the Author
David Burke is Professor and Dean of Research and Development at the College of Health Sciences, University of Sydney.
Read an Excerpt
Cambridge University Press
0521825814 - The Circuitry Of the Human Spinal Cord - Its Role in Motor Control and Movement Disorders - by Emmanuel Pierrot-Deseilligny and David Burke
Excerpt
1
General methodology
The following chapters discuss methods that allow the selective investigation of different spinal pathways. Whatever the pathway investigated, its activation produces changes in the excitability of spinal motoneurones, 'the final common path' in the motor system. A prerequisite for any investigation of changes in the spinal circuitry in human subjects is therefore to be able to assess changes in motoneurone excitability quantitatively, using valid reproducible methods. Several non-invasive methods have been developed, and these are considered in this chapter with their advantages and disadvantages. All are, of course, indirect, and valid conclusions can only be obtained if congruent results are obtained with different methods relying on different principles. All may be, and many have been, used in studies on patients, but here the methodology should be simple and rapid.
This initial chapter is technical and non-specialist readers could bypass it, referring back if they need to clarify how results were obtained or understand the advantages and limitations of a particular technique. However, the chapter is required reading for those who want to understand fully the particular techniques used for the different pathways and how to use those techniques.
The monosynaptic reflex: H reflex and tendon jerk
The 'monosynaptic reflex' forms the basis of the first technique available to investigate spinal pathways in animals and humans. The principle is based on the apparent simplicity of the monosynaptic projection of Ia afferents to homonymous motoneurones. Subsequent studies have shown that the so-called monosynaptic reflex is not as simple as was initially thought. We will consider successively: (ⅰ) the initial findings; (ⅱ) the principles underlying the mono- synaptic reflex testing method; (ⅲ) the basic methodology of the H reflex; (ⅳ) limitations related to mechanisms which can change the size of the reflex by altering its afferent volley; (ⅴ) 'pool problems' related to the input-output relationship within the motoneurone pool.
Initial studies
Animal studies
The monosynaptic reflex depends on the projection of muscle spindle Ia afferents to homonymous motoneurones and was used in the early 1940s as a tool for investigating changes in excitability of the motoneurone pool (Renshaw, 1940; Lloyd, 1941). When used as a test reflex, the monosynaptic reflex allows one to assess the effect on the motoneurone pool of conditioning volleys in peripheral afferents or descending tracts. During the 1940s and early 1950s this method was used to reveal important features of the input to spinal motoneurones. Intracellular recordings later allowed more detailed analysis of the synaptic input to motoneurones in animals (see Baldissera, Hultborn & Illert, 1981), but
Fig. 1.1. Sketch of the pathway of the monosynaptic reflex. Ia afferents from muscle spindle primary endings (dotted line) have monosynaptic projections to α motoneurones (MNs) innervating the corresponding muscle (homonymous MNs). The H reflex is produced by electrical stimulation of Ia afferents, and bypasses muscle spindles. The tendon jerk is elicited by a tap that stretches muscle spindles and therefore also depends on the sensitivity to stretch of primary endings, a property that may be altered by the activity of γ efferents (however, see Chapter 3, pp. 117-18). The pathway of presynaptic inhibition of Ia terminals (see Chapter 8) is represented.
Image not available in HTML version |
interestingly this greater precision did not change the main conclusions that had emerged from the experiments employing the monosynaptic reflex. This suggests that the monosynaptic reflex method produces reliable results.
Human studies
Percutaneous electrical stimulation of the posterior tibial nerve produces a synchronised response in the soleus muscle (Hoffmann, 1918, 1922). This became known as the Hoffmann reflex or H reflex (Magladery & McDougal, 1950). Magladery et al. (1951a) showed that the first motoneurones discharging in the H reflex do so at a latency consistent with a mono- synaptic pathway (see Chapter 2). After the pioneer investigations of Paillard (1955), the H reflex, which is the equivalent of the monosynaptic reflex in animal studies, became the main tool in many motor control investigations and diagnostic studies performed on human subjects (for reviews, see Schieppati, 1987; Burke et al., 1999; Pierrot-Deseilligny & Mazevet, 2000).
Underlying principles
The monosynaptic reflex arc
Pathway
Ia fibres from muscle spindle primary endings have monosynaptic excitatory projections to motoneurones innervating the muscle from which the afferents emanate (homonymous projections, Fig. 1.1). This pathway is responsible for the tendon jerk (see Chapter 2). The H reflex is produced by electrical stimulation of Ia afferents, which have a lower electrical threshold than α motor axons, particularly for stimuli of relatively long duration (see p. 6).
The H reflex, tendon jerk and short-latency spinal stretch reflex
These are all dependent on monosynaptic excitation from homonymous Ia afferents. However, the afferent volleys for these reflexes differ in many respects (cf. Chapter 3): (ⅰ) the electrically induced afferent
Fig. 1.2. Principles of the monosynaptic reflex. (a) Orderly recruitment of motoneurones (MNs) by a given Ia input: the size of the monosynaptic Ia EPSP (upper row) decreases as MN size increases (lower row). The dotted horizontal line represents the threshold for discharge of the MNs. Only the smallest MNs (black) are fired by the test Ia volley, and the excitability of subliminally excited MNs decreases from the smallest to the largest (as indicated by the decreasing tone of grey). (b) Facilitation by an excitatory input. There is summation of the conditioning (thin lines) and test (thick lines) EPSPs. As a result, MNs which had just failed to discharge in the control reflex are raised to firing threshold and the size of the reflex is increased. (c) Inhibition by an inhibitory input. There is summation of the conditioning IPSP (thin line) and of the test EPSP (the test EPSP is also reduced by changes in the membrane conductance, see p. 27). As a result, MNs which had just been recruited in the control reflex cannot be discharged, and the size of the reflex is reduced. Note that the excitability of the MNs in the subliminal fringe of excitation is also modified by the conditioning input. Modified from Pierrot-Deseilligny & Mazevet (2000), with permission.
Image not available in HTML version |
volley for the H reflex bypasses muscle spindles and produces a single synchronous volley in group Ia and Ib afferents; (ⅱ) the tendon tap produces a highly dynamic stretch, which activates mainly muscle spindle primary endings and elicits a prolonged discharge in Ia afferents; (ⅲ) the short-latency Ia spinal stretch reflex is overlapped by a medium-latency response due to a group Ⅱ volley from muscle spindle secondary endings (see Chapter 7).
The orderly recruitment of motoneurones in the monosynaptic reflex
Figure 1.2(a) shows that, in the cat, the size of the test Ia excitatory post-synaptic potential (EPSP) evoked in individual motoneurones by a given afferent volley is larger in small motoneurones supplying slow motor units than in large motoneurones supplying fast units. As a result, motoneurones are recruited in an orderly sequence by the Ia input, from the smallest to the largest, according to Henneman's size prin- ciple (see Henneman & Mendell, 1981). Motoneurones contributing to the human H reflex are recruited in a similar orderly sequence from slow to fast motor units (Buchthal & Schmalbruch, 1970). This orderly recruitment of motoneurones is preserved when they receive a variety of excitatory and inhibitory inputs (though not all, see pp. 18-20), such that facilitation will initially affect those motoneurones that just failed to discharge in the control reflex (dark grey motoneurones in Fig. 1.2(b)) and inhibition will affect those that had just been recruited into the control reflex (largest black motoneurones in Fig. 1.2(a)).
Principles of the monosynaptic reflex method
In the control situation, the test Ia volley elicited by stimulation of constant intensity causes some motoneurones to discharge producing the control test reflex (black motoneurones in Fig. 1.2(a)) and creates EPSPs in other motoneurones which thereby become subliminally excited (grey motoneurones in Fig. 1.2(a)). If motoneurones are now facilitated by a subthreshold conditioning volley, motoneurones that had just failed to fire in the control reflex will discharge when the conditioning and test EPSPs summate (Fig. 1.2(b)). The size of the test reflex will increase. By contrast, if motoneurones receive conditioning inhibitory post-synaptic potentials (IPSPs), the test Ia volley will not be able to discharge the motoneurones that had been recruited last into the control reflex, and the size of the test reflex will be decreased (Fig. 1.2(c)). The method allows one to distinguish between: (ⅰ) conditioning stimuli without effect on the excitability of motoneurones; (ⅱ) those which evoke only subliminal excitation of the motoneurones when applied alone; and (ⅲ) those which inhibit motoneurones. A variant of the method is to compare the amplitude of the reflex in two situations (e.g. 'natural reciprocal inhibition' of the reflex with respect to rest during voluntary contraction of the antagonistic muscle, cf. Chapter 5).
Basic methodology
H reflexes cannot be recorded with equal ease in different motor nuclei (cf. Chapter 2). In most healthy subjects at rest, H reflexes can usually be recorded only from soleus (Hoffmann, 1918), quadriceps (Gassel, 1963), hamstrings (Magladery et al., 1951a) and flexor carpi radialis (FCR) (Deschuytere, Rosselle & DeKeyser, 1976). However, when a weak voluntary contraction is used to potentiate the reflex by raising motoneurone excitability close to firing threshold, H reflexes can be recorded from virtually all limb muscles, if the parent nerve is accessible to electrical stimulation (cf. Burke, Adams & Skuse, 1989; Chapter 2).
General experimental arrangement
Subject's posture
The subject should be comfortably seated in an armchair with the examined limb loosely fixed in a position avoiding stretch of the test muscle (see Hugon, 1973; Burke et al., 1999). Thus, the lower limb is commonly explored with the hip semi-flexed (120°), the knee slightly flexed (160°) and the ankle at 110° plantar flexion. The upper limb is explored with the shoulder in slight abduction (60°), the elbow semi-flexed (110°), and the forearm pronated and supported by the arm of the chair. In patients, recordings can be performed supine, again avoiding stretch on the test muscle.
Awareness
The state of awareness of the subject may modify the amplitude of the H reflex, often in an unpredictable way. The H reflex increases during alertness, at least when the level of attention is high (Bathien & Morin, 1972). Task demands can induce variations in the
Fig. 1.3. Recruitment curve of the H and M waves in the soleus. (a)-(h) Sample EMG responses ((e)-(h)) and sketches of the corresponding volleys in Ia afferents and motor axons ((a)-(d)) when the stimulus intensity is progressively increased. MNs discharged by the Ia volley are black, muscle fibres activated by the H reflex are speckled and those activated by the M wave are hatched. (a) and (e), stimulation (9 mA) activates Ia afferents only and causes MN 'X' to fire in the H reflex. (b) and (f ), stronger stimulation (12 mA) activates more Ia afferents and this causes MNs 'X' and 'Y' to fire in the H reflex, which increases in size. It also elicits a motor volley in the axon of MN 'Z' and an M wave appears in the EMG. The antidromic motor volley in MN 'Z' does not collide with the reflex response, because this MN does not contribute to the reflex. (c) and (g), even stronger stimulation (15 mA) causes MNs 'X' and 'Y' to fire in the H reflex and elicits a motor volley in the axon of MNs 'Z' and 'Y': as a result, an M wave appears in the muscle fibres innervated by MN 'Y'. The antidromic motor volley collides with and eliminates the reflex volley in the axon of MN 'Y', and the H reflex decreases. (d) and (h), yet stronger stimulation (30 mA) produces Mmax, and the H reflex is eliminated by collision with the antidromic motor volley. The vertical dashed line in (e)-(g) indicates the latency of the H reflex. (i), the amplitudes of the H reflex (●) and of the M wave (○) are plotted against stimulus intensity. Modified from Pierrot-Deseilligny & Mazevet (2000), with permission.
Image not available in HTML version |
H reflex related to the particular characteristics of the mental effort required by the task itself (Brunia, 1971). In practice, H reflexes should be recorded in a quiet room, and the influence of the mental effort involved in a difficult motor task should be taken into account. Conversely, the H reflex decreases during the early stages of sleep and is abolished during REM sleep (Hodes & Dement, 1964).
Recording the H reflex
Recording
Reflexes generally appear in the EMG as triphasic waveforms, particularly with soleus where the electrodes are not over the motor point (cf. Fig. 1.3(e)-(g)).
(ⅰ) Bipolar surface electrodes are commonly placed 1.5-2 cm apart over the corresponding muscle belly for recording H and tendon reflexes. For the quadriceps the best place is on the anterior aspect of the thigh, 5-10 cm above the patella over the vastus intermedius. In the forearm, a selective voluntary contraction can be used as a first step to focus the reflex response on the desired motoneurone pool, because during the contraction the reflex discharge can be obtained at lower threshold in the contracting muscle.
(ⅱ) Monopolar recordings, with an 'active' electrode over the mid-belly of the muscle and a 'remote' electrode over its tendon, have been recommended to minimise the effects of changes in geometry of the muscle during voluntary contraction (Gerilovsky, Ysvetinov & Trenkova, 1989). However, these changes are adequately taken into account if the reflex is expressed as a percentage of the maximal M wave (see p. 8) measured under the same conditions. In addition, the more distant the 'remote' electrode, the less likely is the recorded activity to come from only the muscle underlying the 'active' electrode.
Measurement
(ⅰ) Reflex latency is measured to the first deflection of the H wave from baseline, not to the first positive peak of the commonly triphasic waveform (see the vertical dashed line in Fig. 1.3(e)-(g)).
(ⅱ) In practice it makes little difference whether the amplitude or the surface area of the reflex is assessed or whether amplitude is measured for the negative phase only or from negative peak to the following positive peak. Whichever way the H reflex is measured, the same method should be used for the maximal M wave, 'Mmax' (see p. 8), and the amplitude of the H reflex must be expressed as a percentage of Mmax.
Cross-talk
Pick up of the EMG potentials from an adjacent muscle can occur if there is spread of the test stimulus - electrical to another nerve (H reflex), or mechanical to another muscle (tendon jerk) (see Hutton, Roy & Edgerton, 1988). Even if this does not occur, it can still be difficult to be certain that a surface-recorded EMG potential comes exclusively from the underlying muscle rather than a synergist (e.g. responses elicited in the FCR and finger flexors after median nerve stimulation). In addition, responses evoked by a conditioning stimulus may also contaminate the test reflex, e.g. the H reflex in the antagonist FCR when studying reciprocal inhibition from wrist flexors to wrist extensors. Muscle palpation may help recognise inadvertent activation of inappropriate muscles. Another simple way of ensuring that the reflex response originates from the muscle over which it is recorded is to check that it increases during a selective voluntary contraction of that muscle.
Stimulation to elicit the H reflex
H reflexes are produced by percutaneous electrical stimulation of Ia afferents in the parent nerve. The technique is now well codified (see Hugon, 1973; Burke et al., 1999).
Duration of the stimulus
The diameter of Ia afferents is slightly larger than that of α motor axons and their rheobasic threshold is lower, such that it is generally possible, particularly in soleus, to evoke an H reflex with stimuli below motor threshold (1 × MT). The strength-duration curves for motor axons and Ia afferents differ and, as a result, the optimal stimulus duration for eliciting the H reflex is long (1 ms; see Paillard, 1955; Panizza, Nilsson & Hallett, 1989). The stimulus intensity for the threshold H reflex then approaches rheobase for low-threshold Ia afferents, approximately 50% of rheobase for motor axons (Lin et al., 2002).
Unipolar and bipolar stimulation
The best method for ensuring that Ia afferents are excited at lower threshold than motor axons involves placing the cathode over the nerve and the anode on the opposite side of the limb, so that current passes transversely through the nerve. The soleus and quadriceps H reflexes are commonly evoked by monopolar stimulation of the posterior tibial nerve (cathode in the popliteal fossa, anode on the anter- ior aspect of the knee) and the femoral nerve (cathode in the femoral triangle, anode on the poster- ior aspect of the thigh), respectively. However, in areas where there are many nerves, bipolar stimulation may avoid stimulus encroachment upon other nerves: the median nerve (FCR) is so stimulated at the elbow. The same applies to the stimulation of the deep peroneal branch of the common peroneal nerve (tibialis anterior) at the fibular neck and of the sciatic nerve (hamstrings) at the posterior aspect of the thigh. It is generally stated that the cathode should then be placed over the nerve with anode distal (or lateral) to avoid the possibility of anodal block. However, there is little evidence that this is really a problem in practice.
Frequency of stimulation
Because of post-activation depression (see Chapter 2), there is reflex attenuation as stimulus rate is increased above 0.1 Hz. This attenuation requires at least 10 s to subside completely, but its effects are sufficiently small after 3-4 s to allow testing at 0.2-0.3 Hz. Use of these frequencies constitutes a compromise between reflex depression and the necessity to collect a large number of responses because of reflex variability. During a background contraction of the tested muscle, the attenuation with increasing stimulus repetition rate is reduced or even abolished (cf. Chapter 2).
Magnetic stimulation
The H reflex may also be evoked by magnetic stimulation of the parent nerve (or nerve root) and appears with the same latency as with electrical stimulation (Zhu et al., 1992). One advantage of magnetic stimulation is the ease with which an H reflex can be elicited from deep nerves, such as the sciatic nerve in the thigh or the sacral nerve roots, which are difficult to access with percutaneous electrical stimulation unless needle electrodes are inserted (Abbruzzese et al., 1985). However, with magnetic stimulation, the threshold for the H reflex is usually higher than that for the M wave. This difference is probably due to the extreme brevity (∼0.05 ms) of the effective stimulus produced by magnetic stimulation, a stimulus duration that favours motor axons with respect to Ia afferents (Panizza et al., 1992).
H and M recruitment curve
The recruitment curve
As the intensity of the electrical stimulus to the posterior tibial nerve is increased, there is initially a progressive increase in amplitude of the soleus reflex due to the stronger Ia afferent volley (Fig. 1.3(a), (b), (e), (f )). When motor threshold is reached, the short-latency direct motor response (M wave) appears in the EMG due to stimulation of motor axons ((b) and (f )). Further increases in the intensity of the test stimulus cause the M wave to increase and the H reflex to decrease ((c) and (g)). Finally, when the direct motor response is maximal, the reflex response is completely suppressed ((d) and (h)). This is because the antidromic motor volley set up in motor axons collides with and eliminates the H reflex response (Hoffmann, 1922, Fig. 1.3(d)). Note that, when it first appears in the EMG, the M response involves axons of the largest motoneurones (e.g. MN 'Z' in Fig. 1.3(b) and (f )), which have a high threshold for recruitment into the H reflex. Because they are not activated in the reflex, stimulation of these motor axons does not interfere with the reflex response. The variations of the H and M responses with the test stimulus intensity can be plotted as the recruitment curve of Fig. 1.3(i). Because of the orderly recruitment of motoneurones (see pp. 3-4), the sensitivity of the reflex to facilitation and inhibition depends on the last motoneurones recruited by the test volley (as long as the reflex is not on the descending limb of the recruitment curve, see below).
Maximal M wave (Mmax)
Mmax is evoked by the stimulation of all motor axons and provides an estimate of the response of the entire motoneurone pool. This estimate is actually an overestimate, because the necessarily strong stimulus will produce EMG activity in synergists in addition to the test muscle. Accordingly, the Mmax following median nerve stimulation at the elbow comes from the FCR, finger flexors and pronator teres. Ignoring this issue, Mmax should always be measured in the same experiment with the same recording electrode placement because: (ⅰ) comparing it with the reflex response provides an estimate of the proportion of the motoneurone pool discharging in the reflex; (ⅱ) expressing the reflex as a percentage of Mmax enables one to control for changes in muscle geometry due to changes in muscle length or contraction; (ⅲ) expressing the test reflex as a percentage of Mmax allows the investigator to be sure that the test reflex remains within the 'linear' range of the input/output relationship for the motoneurone pool (i.e. between ∼10 and 60% of Mmax for the soleus H reflex, see pp. 16-18).
The test reflex should not be on the descending limb of the recruitment curve
This is because the component of the H reflex seen in the EMG is generated by low-threshold motoneurones, which are insensitive to excitation or inhibition. Small motoneurones innervating slow motor units are first recruited in the H reflex (see pp. 3-4), whereas electrical stimulation will first activate motor axons of large diameter from high-threshold motoneurones. As a result, on the descending limb of the recruitment curve, the reflex response seen in the EMG will be produced by small motoneurones, in which the collision in motor axons has not taken place. The reflex response in the fastest motor units of the H reflex, i.e. those that were last recruited into the reflex and are thus sensitive to excitation and inhibition, will be eliminated by collision with the antidromic motor volley (Fig. 1.3(c) and (g)) (see Pierrot-Deseilligny & Mazevet, 2000).
Monitoring the stability of the stimulation conditions
If the H reflex is performed during a manoeuvre that can alter the stimulating conditions (e.g. muscle contraction, stance or gait), it is necessary to ensure that changes in the test H reflex are not due to a change in the position of the stimulating electrode. The reproducibility of a M wave can then be used to monitor the stability of the stimulation. To that end, stimulation should be adjusted to produce a small M wave in addition to the H reflex. If there is need for a test response without a M wave, the stability of stimulation can be monitored by alternating the test stimulus with a stimulus evoking a M wave through the same electrode. This procedure raises questions about the acceptable range of variability of the M wave in such studies. Some authors have used a range of ±10% of Mmax, but this is a large range when compared to the changes expected in the H reflex, and changes not exceeding ±10% of the recorded M wave, not ±10% of Mmax, are recommended. It should be realised that, during experiments involving a voluntary or postural contraction of the tested muscle, there will be changes in axonal excitability unrelated to stability of the stimulating conditions (Vagg et al., 1998), and there will inevitably be some variability in the M wave from trial to trial.
Recruitment curves in other muscles
The recruitment curves for the quadriceps and FCR H reflexes are similar. However, the threshold of the M and H responses of FCR and quadriceps are generally closer than in soleus.
Tendon jerk
In proximal muscles (e.g. biceps and triceps brachii), the H reflex is difficult to record at rest without the M wave, and it then appears merged into the end of the M wave. For routine testing, it may be more convenient to test the excitability of these motoneurone pools using tendon reflexes. An electromagnetic hammer (such as a Brüel and Kjaer shaker, Copenhagen, Denmark) will produce reproducible transient tendon percussion. In healthy subjects at rest, a tendon jerk reflex can be elicited in the soleus, quadriceps, biceps femoris, semitendinosus, biceps and triceps brachii, FCR, extensor carpi radialis (ECR) and the masseter. Use of the tendon jerk introduces two complications.
Delay due to the tendon tap
The tendon tap introduces a delay, and in the soleus, the afferent volley for the tendon jerk will reach motoneurones ∼5 ms later than the electrically induced volley producing the H reflex (cf. Chapter 2). An estimate of the central delay of the effect of a conditioning volley on a test tendon jerk may be obtained by comparing the first interstimulus interval (ISI) at which this effect occurs to the first ISI at which a heteronymous monosynaptic Ia volley delivered to the same nerve facilitates the tested motoneurones (see Mazevet & Pierrot-Deseilligny, 1994). An example would be the group Ia projection from median-innervated forearm muscles to biceps and triceps motoneurones.
Fusimotor drive
The amplitude of the reflex response produced by tendon percussion may depend on the level of γ drive directed to muscle spindle primary endings of the tested muscle (see Fig. 1.1. Accordingly, it has been argued that differences in the behaviour of H and tendon jerk reflexes reflect the involvement of γ drive in the tendon jerk (e.g. see Paillard, 1955). This belief has been called into question because H and tendon jerk reflexes differ in a number of other respects, as discussed in detail in Chapter 3. Of greater importance could well be the effects on the spindle response to percussion of the thixotropic properties of intrafusal fibres (see Chapter 3).
Random alternation of control and conditioned reflexes
In most investigations, the monosynaptic reflex is used as a test reflex to assess the effect of conditioning volleys on the motoneurone pool. The size of the reflex is compared in the absence (control reflex) and in the presence (conditioned reflex) of the conditioning volley. Control and conditioned reflexes should be randomly alternated, because: (ⅰ) this avoids the possibility of the subject voluntarily or involuntarily predicting the reflex sequence; and (ⅱ) regular alternation produces erroneously large results (Fournier, Katz & Pierrot-Deseilligny, 1984), possibly due to post-activation depression (see Pierrot-Deseilligny & Mazevet, 2000).
Estimate of the central delay of a conditioning effect. Time resolution of the method
It is essential to estimate the central delay of an effect in order to characterise the neural pathway activated by a conditioning stimulus as mono-, di-, or poly-synaptic. This can be done by comparing the earliest conditioning-test interval at which the test reflex is modified with the interval estimated for the simultaneous arrival of the conditioning and test volleys at spinal level. The greater the difference between these two values the longer the intraspinal circuit, and this could be because more synapses are involved. It should be noted that the H reflex method under- estimates the true central delay. For example, despite the extra 0.8 ms due to the interneurone interposed in the pathway of disynaptic reciprocal Ia inhibition, the earliest conditioning-test interval with inhibition corresponds to the simultaneous arrival of the two volleys at spinal level (Chapter 5). This is due to two reasons (Fig. 1.4).
PSPs in individual motoneurones
The rise time of the EPSP is sufficiently long that the discharge of the last recruited motoneurones evoked by the monosynaptic input will not occur before the arrival of a disynaptic IPSP. This is so even though the synaptic delay at the interneurone delays the onset of the IPSP by 0.5-1.0 ms relative to the beginning of the monosynaptic EPSP (see Matthews, 1972, and Fig. 1.4(a)). In addition, an EPSP elicited by a conditioning volley entering the spinal cord after the test volley may summate with the decay phase of the test Ia EPSP and cause the motoneurone to discharge at a 'too early' ISI.
Motoneurones do not discharge at the same time in the test reflex
Even in the cat there is 0.5 ms between the firing of the first and last recruited motoneurones contributing to
Fig. 1.4. A disynaptic IPSP can inhibit the monosynaptic reflex. (a) Post-synaptic (PSP) potentials in an individual motoneurone (MN): when volleys eliciting a monosynaptic Ia EPSP and a disynaptic IPSP enter the spinal cord simultaneously, the rise time of the EPSP in individual MNs allows the spike in the last recruited MNs to be inhibited by the IPSP, even though the latter does not begin until 0.5-1.0 ms after the beginning of the EPSP. (b) MNs contributing to the H reflex do not discharge simultaneously in the test reflex. Thus, a disynaptic IPSP elicited by a conditioning volley entering the spinal cord at the same time as the test monosynaptic Ia volley may inhibit the last spikes (thin interrupted lines) contributing to the monosynaptic reflex discharge, while the first spikes (thick continuous lines) are not modified. Adapted from Matthews (1972) (a), and Araki, Eccles & Ito (1960) (b), with permission.
Image not available in HTML version |
the monosynaptic reflex (Araki, Eccles & Ito, 1960). In human subjects, where the afferent pathway is longer and the conduction velocity of Ia afferents slower, this interval has been estimated at 1.5 ms for the quadriceps H reflex (Fournier et al., 1986) and ∼2 ms for the soleus H reflex (Burke, Gandevia & McKeon, 1984). There are differences in the rise-times of mechanically and electrically evoked EPSPs (∼10 ms for tendon percussion; ∼2 ms for the electrically evoked volley), but this is not obvious in the reflex EMG potentials because the axons of the last recruited motoneurones have a more rapid conduction velocity than those first recruited. Figure 1.4(b) shows that, because of the desynchronisation at spinal level, the last individual spikes contributing to the monosynaptic test reflex discharge can be inhibited by a disynaptic IPSP elicited by a conditioning volley entering the spinal cord at the same time as the monosynaptic test volley.
The recovery cycle of the H reflex
The recovery cycle of the H reflex investigates the time course of the changes in the H reflex after a conditioning reflex for conditioning-test intervals up to 1-2 s. Such studies were in vogue in the 1950-1960s (Magladery et al., 1951b, 1952; Paillard, 1955). However, the recovery cycle is no longer employed, because it results from too many phenomena to be of practical use. Factors that could alter the test reflex include changes in excitability of Ia afferents (see below), post-activation depression (cf. pp. 13-14), presynaptic inhibition of Ia terminals activated by the conditioning volley (cf. Chapter 8), afterhyperpolarisation and recurrent inhibition of motoneurones (cf. Chapter 4), muscle spindle receptor unloading by the conditioning twitch (cf. Chapter 3), Golgi tendon organ activation by the conditioning twitch (cf. Chapter 6), and effects mediated by long loops (Táboríková & Sax, 1969).
© Cambridge University Press