Risk Assessment of Power Systems: Models, Methods, and Applications, Second Edition fills the gap between risk theory and real-world application. Author Wenyuan Li is a leading authority on power system risk and has more than twenty-five years of experience in risk evaluation. This book offers real-world examples to help readers learn to evaluate power system risk during planning, design, operations, and maintenance activities.
Some of the new additions in the Second Edition include:
- New research and applied achievements in power system risk assessment
- A discussion of correlation models in risk evaluation
- How to apply risk assessment to renewable energy sources and smart grids
- Asset management based on condition monitoring and risk evaluation
- Voltage instability risk assessment and its application to system planning
The book includes theoretical methods and actual industrial applications. It offers an extensive discussion of component and system models, applied methods, and practical examples, allowing readers to effectively use the basic concepts to conduct risk assessments for power systems in the real world. With every original chapter updated, two new sections added, and five entirely new chapters included to cover new trends, Risk Assessment of Power Systems is an essential reference.
Risk Assessment of Power Systems: Models, Methods, and Applications, Second Edition fills the gap between risk theory and real-world application. Author Wenyuan Li is a leading authority on power system risk and has more than twenty-five years of experience in risk evaluation. This book offers real-world examples to help readers learn to evaluate power system risk during planning, design, operations, and maintenance activities.
Some of the new additions in the Second Edition include:
- New research and applied achievements in power system risk assessment
- A discussion of correlation models in risk evaluation
- How to apply risk assessment to renewable energy sources and smart grids
- Asset management based on condition monitoring and risk evaluation
- Voltage instability risk assessment and its application to system planning
The book includes theoretical methods and actual industrial applications. It offers an extensive discussion of component and system models, applied methods, and practical examples, allowing readers to effectively use the basic concepts to conduct risk assessments for power systems in the real world. With every original chapter updated, two new sections added, and five entirely new chapters included to cover new trends, Risk Assessment of Power Systems is an essential reference.
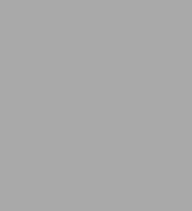
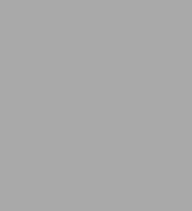
eBook
Available on Compatible NOOK devices, the free NOOK App and in My Digital Library.
Related collections and offers
Overview
Risk Assessment of Power Systems: Models, Methods, and Applications, Second Edition fills the gap between risk theory and real-world application. Author Wenyuan Li is a leading authority on power system risk and has more than twenty-five years of experience in risk evaluation. This book offers real-world examples to help readers learn to evaluate power system risk during planning, design, operations, and maintenance activities.
Some of the new additions in the Second Edition include:
- New research and applied achievements in power system risk assessment
- A discussion of correlation models in risk evaluation
- How to apply risk assessment to renewable energy sources and smart grids
- Asset management based on condition monitoring and risk evaluation
- Voltage instability risk assessment and its application to system planning
The book includes theoretical methods and actual industrial applications. It offers an extensive discussion of component and system models, applied methods, and practical examples, allowing readers to effectively use the basic concepts to conduct risk assessments for power systems in the real world. With every original chapter updated, two new sections added, and five entirely new chapters included to cover new trends, Risk Assessment of Power Systems is an essential reference.
Product Details
ISBN-13: | 9781118843222 |
---|---|
Publisher: | Wiley |
Publication date: | 02/19/2014 |
Series: | IEEE Press Series on Power and Energy Systems |
Sold by: | JOHN WILEY & SONS |
Format: | eBook |
Pages: | 560 |
File size: | 17 MB |
Note: | This product may take a few minutes to download. |
About the Author
Read an Excerpt
Risk Assessment of Power Systems
Models, Methods, and ApplicationsBy Wenyuan Li
John Wiley & Sons
Copyright © 2005 Institute of Electrical and Electronics Engineers, Inc.All right reserved.
ISBN: 0-471-63168-X
Chapter One
INTRODUCTION1.1 RISK IN POWER SYSTEMS
There is considerable overlap in the words "risk" and "reliability." In this book, it is assumed that the two words have the identical implication. They are the two facets of the same fact. Higher risk means lower reliability, and vice versa. Risk management has a wide-ranging content. The intent of the book is to discuss the models, methods, and applications of risk assessment in physical power systems. Risks associated with business, finance, and life safety are not included in the discussion.
The probabilistic behavior of power systems is the root origin of risk. Random failures of system equipment are generally outside the control of power system personnel. Loads always have uncertainties and it is impossible to obtain an exact load forecast. Energy exports and imports under the deregulation environment depend on the volatile power market. Consequences of power failures range from electricity interruptions in local areas to a possible widespread blackout. Economic impacts due to supply interruptions are not restricted to loss of revenue by the utility or loss of energy utilization by the customer butinclude indirect costs imposed on society and the environment. Risk assessment has become a challenge and an essential commitment in the power utility industry today.
Risk management includes at least the following three tasks:
1. Performing quantitative risk evaluation
2. Determining measures to reduce risk
3. Justifying an acceptable risk level
The purpose of quantitative evaluation is to create the indices representing system risk. A dictionary definition of risk is "the probability of loss or damage to human beings or assets." This definition can be used in general cases. However, a comprehensive risk index should not contain only the probability but a combination of probability and consequence. In other words, the risk evaluation of power systems should recognize not only the likelihood of failure events but also the severity and degree of their consequences. Utilities have dealt with system risks for a long time. The criteria and methods first used in practical applications were all deterministically based, such as the percentage reserve in generation capacity planning and the single-contingency principle in transmission planning. The deterministic criteria have served the power industry for years. The basic weakness is that they do not respond to the probabilistic nature of power system behavior, load variation, and component failures.
A measure to reduce system risk is generally associated with enhancement of the system. In order to determine a rational measure, both the impact of the measure on risk reduction and the cost needed to implement it should be quantified. A probabilistic economic analysis is usually required. In risk management, an important concept to be appreciated is that zero risk can never be reached since random failure events are uncontrollable. In many cases, a decision has to be made to accept a risk as long as it can be technically and financially justified. Selecting a rational measure to reduce risks or accepting a risk level is a decision-making process. It should be recognized that on the one hand, quantitative risk evaluation is the basis of this process, and on the other hand, the process is more than risk evaluation and requires technical, economic, societal, and environmental assessments.
The risk assessment of power systems can be applied to all the areas in electric power utilities, including:
Quantified reliability evaluation in generation, transmission, and distribution systems
Probabilistic criteria in system planning and operation
Compromise between the system risk and the economic benefit in a decision-making process
Equipment aging failure management
Spare equipment strategy
Reliability-centered maintenance
Load-side risk management
Performance-based rate policy
Operation-risk monitoring
Interruption damage cost assessment
Risk management and quantified risk assessment have become ever-increasingly important since the power industry entered the deregulation era. The new competition environment forces utilities to plan and operate their systems closer to the limit. The stressed operation conditions have led to deterioration in system reliability. In fact, a lot of power-outage events have occurred across the world in the past years. According to an EPRI (Electric Power Research Institute) report based on the national survey in all business sectors, the U.S. economy alone is losing between $104 and $164 billion a year due to power system outages. Severe power outage events have happened frequently in recent years. For instance, a major system disturbance separated the Western Electricity Coordinating Council (WECC) system in the west of North America into four islands on August 10, 1996, interrupting electricity service to 7.5 million customers for a period of up to nine hours. The 1998 blackout at the Auckland central business district in New Zealand impacted 30 square blocks of the downtown area for about two months, resulting in lawsuits totaling $600 million against the utility. On August 14, 2003, the massive blackout in the east of North America covered eight states in the United States and two provinces in Canada, bringing about 50 million people into darkness for periods ranging from one to several days. These severe power outages let us realize that the single-contingency criterion (the N-1 principle) that has been used for many years in the power industry may not be sufficient to preserve a reasonable system reliability level. However, it is also commonly recognized that no utility can financially justify the N-2 or N-3 principle in power system planning. Obviously, one alternative is to bring risk management into the practice in planning, design, operation, and maintenance, keeping system risk within an acceptable range. On the other hand, the customers of the power industry have become more and more knowledgable about electric power systems. They understand that it is impossible to expect 100% continuity in the power supply without any risk of outages. However, they have the right to know the risk level, including information on how often, for how long, and how severely a power interruption event can happen to them on the average. To answer this question is one of the objectives of power system risk assessment.
1.2 BASIC CONCEPTS OF POWER SYSTEM RISK ASSESSMENT
1.2.1 System Risk Evaluation
Power system risk evaluation is generally associated with the following four tasks:
1. Determining component outage models
2. Selecting system states and calculating their probabilities
3. Evaluating the consequences of selected system states
4. Calculating the risk indices
A power system consists of many components, including generators, transmission lines, cables, transformers, breakers, switches, and a variety of reactive power source equipment. Component outages are the root cause of a system failure state. The first task in system risk evaluation is to determine component outage models. Component failures are classed into two categories: independent and dependent outages. Each category can be further classified according to the outage modes. In most cases, only repairable forced outages are considered, whereas in some cases, planned outages are also modeled. Aging failures have not been incorporated into the traditional risk evaluation. This book presents a modeling approach to aging failures and demonstrates examples of its application.
The second task is to select system failure states and calculate their probabilities. There are two basic methods for selecting a system state: state enumeration and Monte Carlo simulation. Both methods have merits and demerits. In general, if complex operating conditions are not considered and/or the failure probabilities of components are quite small, the state enumeration techniques are more efficient. When complex operating conditions are involved and/or the number of severe events is relatively large, Monte Carlo methods are often preferable.
The third task is to perform the analysis for system failure states and assess their consequences. Depending on the system under study, the analysis could be associated with the simple power balance, or the connectivity identification of a network configuration, or the complex calculation process, including the power flow, optimal power flow, or even transient and voltage-stability evaluation.
As mentioned earlier, risk is a combination of probability and consequence. With the information obtained in the second and third tasks, an index that truly represents system risk can be created. There are many possible risk indices for different purposes. Most of them are basically the expected value of a random variable, although a probability distribution can be calculated in some cases. It is important to appreciate that the expected value is not a deterministic parameter. It is the longrun average of the phenomenon under study. The expected indices serve as the risk indicators that reflect various factors, including component capacities and outages, load profiles and forecast uncertainties, system configurations and operational conditions, and so on.
According to system state analysis, power system risk assessment can be divided into two basic aspects: system adequacy and system security. Adequacy relates to the existence of sufficient facilities within the system to satisfy consumer load demand and system operational constraints. Adequacy is therefore associated with the static conditions that do not include system dynamic and transient processes. Security relates to the ability of the system to respond to dynamic and transient disturbances arising within the system. Security is therefore associated with the response of the system to whatever perturbations it is subject to. Normally, security evaluation requires the analysis of dynamic, transient, or voltage stability in the system. It should be pointed out that most of the risk evaluation techniques that have been used in the actual applications of utilities are in the domain of adequacy assessment. Some ideas for security assessment have been addressed recently. However, the practical application in this area is limited. Another fact is that most of the risk indices used in risk evaluation are inadequacy indices, not overall risk indices. The system indices that are based on historical outage statistics encompass the effect of both inadequacy and insecurity. It is important to recognize this fundamental difference in actual engineering applications.
A power system includes the three fundamental functions of generation, transmission (including substation), and distribution. Traditionally, the three functional zones are included in one utility. As reform in the power industry proceeds, the three functional zones have been gradually separated to form organizationally independent generation, transmission, and distribution companies in many countries. In either case, risk assessment can be, and is, conducted in each of these functional zones. The risk evaluation for an overall system, including generation, transmission, and distribution, is impractical because such a system is too enormous to handle in terms of the existing computing capacity and accuracy requirements. On the one hand, the calculation modeling and algorithms are quite different for the risk evaluation of a generation, transmission, substation, distribution system. On the other hand, many techniques have been successfully developed to perform the risk evaluation for composite generation and transmission systems or composite transmission and substation systems. In the case of a large-scale transmission system, it is reasonable to limit the study to an area or subsystem. Doing so can provide more realistic results than evaluating the whole system. This is due to the fact that a change or reinforcement in the network may considerably affect a local area but have little impact on remote parts of the system. The contribution to the overall reliability of a large system due to a local line addition or reconfiguration may be so small that it is masked by computational errors and, consequently, cannot be reflected in the risk change of the whole system. This contribution, however, can be a relatively large portion of the risk change in the local area.
Generally, it is necessary to assess the relative benefits of different alternatives, including the option of doing nothing. The level of analysis need not be any more complex than that which enables the relative merits to be assessed. The ability to include a high degree of precision in calculations should never override the inherent uncertainty in the data. An absolute risk index, although an ideal objective, is virtually impossible to evaluate. This does not weaken the necessity to objectively assess the relative merits of alternative schemes. This is an important point to be appreciated in power system risk evaluation.
1.2.2 Data in Risk Evaluation
The reliability data required in power system risk evaluation are the parameters of component outage models. They are basically calculated from historical statistics, although an engineering judgment based on individual equipment assessments is also used in some special cases. Collecting suitable data is at least as essential as developing risk evaluation methods.
The data requirements should reflect the need for risk assessments. The data must be sufficiently comprehensive to ensure that an evaluation method can be applied, but restrictive enough to ensure that unnecessary data are not collected. For the simple models, the data relates to the two main processes of component behavior, namely, the failure process and the restoration process. For more complex models, the data are associated with the transition rates between various states.
The quality of data is an important factor to consider in data collection. The usual saying of "garbage in and garbage out" refers to the fact that if the quality of data cannot be guaranteed, the results of risk evaluation will not make any sense. Outage statistics constitute a huge data pool and some bad or invalid records cannot be fully avoided in any database. Data processing is necessary to filter out bad data. A parameter estimation procedure is needed to acquire the input data of risk evaluation from raw statistics. This requires the suitable design of statistical data modeling.
Another characteristic of reliability data is its dynamic feature. The volume of outage records will increase with the time and, therefore, the average failure frequency and repair time for a piece of equipment or an equipment group will change from year to year.
Continues...
Excerpted from Risk Assessment of Power Systems by Wenyuan Li Copyright © 2005 by Institute of Electrical and Electronics Engineers, Inc. . Excerpted by permission.
All rights reserved. No part of this excerpt may be reproduced or reprinted without permission in writing from the publisher.
Excerpts are provided by Dial-A-Book Inc. solely for the personal use of visitors to this web site.
Table of Contents
Preface xixPreface to the First Edition xxi
1 Introduction 1
1.1 Risk in Power Systems 1
1.2 Basic Concepts of Power System Risk Assessment 4
1.2.1 System Risk Evaluation 4
1.2.2 Data in Risk Evaluation 6
1.2.3 Unit Interruption Cost 7
1.3 Outline of the Book 9
2 Outage Models of System Components 15
2.1 Introduction 15
2.2 Models of Independent Outages 16
2.2.1 Repairable Forced Failure 17
2.2.2 Aging Failure 18
2.2.3 Nonrepairable Chance Failure 24
2.2.4 Planned Outage 24
2.2.5 Semiforced Outage 27
2.2.6 Partial Failure Mode 28
2.2.7 Multiple Failure Mode 30
2.3 Models of Dependent Outages 31
2.3.1 Common-Cause Outage 31
2.3.2 Component-Group Outage 36
2.3.3 Station-Originated Outage 37
2.3.4 Cascading Outage 39
2.3.5 Environment-Dependent Failure 40
2.4 Conclusions 42
3 Parameter Estimation in Outage Models 45
3.1 Introduction 45
3.2 Point Estimation on Mean and Variance of Failure Data 46
3.2.1 Sample Mean 46
3.2.2 Sample Variance 48
3.3 Interval Estimation on Mean and Variance of Failure Data 49
3.3.1 General Concept of Confidence Interval 49
3.3.2 Confidence Interval of Mean 50
3.3.3 Confidence Interval of Variance 53
3.4 Estimating Failure Frequency of Individual Components 54
3.4.1 Point Estimation 54
3.4.2 Interval Estimation 55
3.5 Estimating Probability from a Binomial Distribution 56
3.6 Experimental Distribution of Failure Data and its Test 57
3.6.1 Experimental Distribution of Failure Data 58
3.6.2 Test of Experimental Distribution 59
3.7 Estimating Parameters in Aging Failure Models 60
3.7.1 Mean Life and its Standard Deviation in the Normal Model 61
3.7.2 Shape and Scale Parameters in the Weibull Model 63
3.7.3 Example 66
3.8 Conclusions 70
4 Elements of Risk Evaluation Methods 73
4.1 Introduction 73
4.2 Methods for Simple Systems 74
4.2.1 Probability Convolution 74
4.2.2 Series and Parallel Networks 75
4.2.3 Minimum Cutsets 78
4.2.4 Markov Equations 79
4.2.5 Frequency-Duration Approaches 81
4.3 Methods for Complex Systems 84
4.3.1 State Enumeration 84
4.3.2 Nonsequential Monte Carlo Simulation 87
4.3.3 Sequential Monte Carlo Simulation 89
4.4 Correlation Models in Risk Evaluation 91
4.4.1 Correlation Measures 92
4.4.2 Correlation Matrix Methods 93
4.4.3 Copula Functions 95
4.5 Conclusions 102
5 Risk Evaluation Techniques for Power Systems 105
5.1 Introduction 105
5.2 Techniques Used in Generation-Demand Systems 106
5.2.1 Convolution Technique 106
5.2.2 State Sampling Method 110
5.2.3 State Duration Sampling Method 112
5.3 Techniques Used in Radial Distribution Systems 114
5.3.1 Analytical Technique 114
5.3.2 State Duration Sampling Method 117
5.4 Techniques Used in Substation Configurations 118
5.4.1 Failure Modes and Modeling 119
5.4.2 Connectivity Identification 121
5.4.3 Stratified State Enumeration Method 123
5.4.4 State Duration Sampling Method 127
5.5 Techniques Used in Composite Generation and Transmission Systems 129
5.5.1 Basic Procedure 130
5.5.2 Component Failure Models 131
5.5.3 Load Curve Models 131
5.5.4 Contingency Analysis 133
5.5.5 Optimization Models for Load Curtailments 135
5.5.6 State Enumeration Method 138
5.5.7 State Sampling Method 139
5.6 Conclusions 141
6 Application of Risk Evaluation to Transmission Development Planning 143
6.1 Introduction 143
6.2 Concept of Probabilistic Planning 144
6.2.1 Basic Procedure 144
6.2.2 Cost Analysis 145
6.2.3 Present Value 146
6.3 Risk Evaluation Approach 146
6.3.1 Risk Evaluation Procedure 147
6.3.2 Risk Cost Model 147
6.4 Example 1: Selecting the Lowest-Cost Planning Alternative 149
6.4.1 System Description 149
6.4.2 Planning Alternatives 151
6.4.3 Risk Evaluation 152
6.4.4 Overall Economic Analysis 155
6.4.5 Summary 157
6.5 Example 2: Applying Different Planning Criteria 158
6.5.1 System and Planning Alternatives 158
6.5.2 Study Conditions and Data 159
6.5.3 Risk and Risk Cost Evaluation 161
6.5.4 Overall Economic Analysis 163
6.5.5 Summary 166
6.6 Conclusions 167
7 Application of Risk Evaluation to Transmission Operation Planning 169
7.1 Introduction 169
7.2 Concept of Risk Evaluation in Operation Planning 170
7.3 Risk Evaluation Method 173
7.4 Example 1: Determining the Lowest-Risk Operation Mode 175
7.4.1 System and Study Conditions 175
7.4.2 Assessing Impacts of Load Transfer 177
7.4.3 Comparing Different Reconfigurations 177
7.4.4 Selecting Operation Mode under the N−2 Condition 179
7.4.5 Summary 181
7.5 Example 2: A Simple Case by Hand Calculation 181
7.5.1 Basic Concept 181
7.5.2 Case Description 182
7.5.3 Study Conditions and Data 183
7.5.4 Risk Evaluation 185
7.5.5 Summary 188
7.6 Conclusions 188
8 Application of Risk Evaluation to Generation Source Planning 191
8.1 Introduction 191
8.2 Procedure of Reliability Planning 192
8.3 Simulation of Generation and Risk Costs 193
8.3.1 Simulation Approach 193
8.3.2 Minimization Cost Model 194
8.3.3 Expected Generation and Risk Costs 195
8.4 Example 1: Selecting Location and Size of Cogenerators 196
8.4.1 Basic Concept 196
8.4.2 System and Cogeneration Candidates 197
8.4.3 Risk Sensitivity Analysis 199
8.4.4 Maximum Benefit Analysis 201
8.4.5 Summary 205
8.5 Example 2: Making a Decision to Retire a Local Generation Plant 205
8.5.1 Case Description 206
8.5.2 Risk Evaluation 206
8.5.3 Total Cost Analysis 208
8.5.4 Summary 210
8.6 Conclusions 210
9 Application of Risk Evaluation to Selecting Substation Configurations 211
9.1 Introduction 211
9.2 Load Curtailment Model 212
9.3 Risk Evaluation Approach 215
9.3.1 Component Failure Models 215
9.3.2 Procedure of Risk Evaluation 215
9.3.3 Economic Analysis Method 216
9.4 Example 1: Selecting Substation Configuration 217
9.4.1 Two Substation Configurations 217
9.4.2 Risk Evaluation 218
9.4.3 Economic Analysis 222
9.4.4 Summary 223
9.5 Example 2: Evaluating Effects of Substation Configuration Changes 223
9.5.1 Simplified Model for Evaluating Substation Configurations 223
9.5.2 Problem Description 224
9.5.3 Risk Evaluation 227
9.5.4 Summary 228
9.6 Example 3: Selecting Transmission Line Arrangement Associated with Substations 229
9.6.1 Description of Two Options 229
9.6.2 Risk Evaluation and Economic Analysis 230
9.6.3 Summary 233
9.7 Conclusions 233
10 Application of Risk Evaluation to Renewable Energy Systems 235
10.1 Introduction 235
10.2 Risk Evaluation of Wind Turbine Power Converter System (WTPCS) 237
10.2.1 Basic Concepts 237
10.2.2 Power Losses and Temperatures of WTPCS Components 238
10.2.3 Risk Evaluation of WTPCS 240
10.2.4 Case Study 245
10.2.5 Summary 251
10.3 Risk Evaluation of Photovoltaic Power Systems 251
10.3.1 Two Basic Structures of Photovoltaic Power Systems 251
10.3.2 Risk Parameters of Photovoltaic Inverters 254
10.3.3 Risk Evaluation of Photovoltaic Power System 258
10.3.4 Case Study 263
10.3.5 Summary 270
10.4 Conclusions 272
11 Application of Risk Evaluation to Composite Systems with Renewable Sources 275
11.1 Introduction 275
11.2 Risk Assessment of a Composite System with Wind Farms and Solar Power Stations 276
11.2.1 Probability Models of Renewable Sources and Bus Load Curves 276
11.2.2 Multiple Correlations among Renewable Sources and Bus/Regional Loads 279
11.2.3 Risk Assessment Considering Multiple Correlations 282
11.2.4 Case Study 283
11.2.5 Summary 295
11.3 Determination of Transfer Capability Required by Wind Generation 296
11.3.1 System, Conditions, and Method 296
11.3.2 Wind Generation Model 298
11.3.3 Equivalence of Wind Power in Generation Systems 299
11.3.4 Transfer Capability Required by Wind Generation 303
11.3.5 Summary 309
11.4 Conclusions 310
12 Risk Evaluation of Wide Area Measurement and Control System 313
12.1 Introduction 313
12.2 Hierarchical Structure and Failure Analysis of WAMCS 314
12.2.1 Hierarchical Structure of WAMCS 314
12.2.2 Failure Analysis Technique for WAMCS 315
12.3 Risk Evaluation of Phasor Measurement Units 317
12.3.1 Markov State Models of PMU Modules 317
12.3.2 Equivalent Two-State Model of PMU 324
12.4 Risk Evaluation of Regional Communication Networks in WAMCS 325
12.4.1 Classification of Regional Communication Networks 325
12.4.2 Survival Mechanisms of Regional Networks 328
12.4.3 Risk Evaluation in Two Survival Mechanisms 329
12.4.4 Equivalent Two-State Model of a Regional Communication Network 334
12.5 Risk Evaluation of Backbone Network in WAMCS 335
12.5.1 Equivalent Risk Model of Backbone Communication Network 336
12.5.2 Risk Evaluation of Optic Fiber System 337
12.6 Numerical Results 343
12.6.1 Risk Indices of PMU 343
12.6.2 Risk Indices of Regional Communication Networks 345
12.6.3 Risk Indices of the Backbone Communication Network 347
12.6.4 Risk Indices of Overall WAMCS 348
12.7 Conclusions 349
13 Reliability-Centered Maintenance 351
13.1 Introduction 351
13.2 Basic Tasks in RCM 352
13.2.1 Comparison between Maintenance Alternatives 352
13.2.2 Lowest-Risk Maintenance Scheduling 353
13.2.3 Predictive Maintenance versus Corrective Maintenance 353
13.2.4 Ranking Importance of Components 354
13.3 Example 1: Transmission Maintenance Scheduling 355
13.3.1 Procedure of Transmission Maintenance Planning 355
13.3.2 Description of the System and Maintenance Outage 357
13.3.3 The Lowest-Risk Schedule of the Cable Replacement 358
13.3.4 Summary 359
13.4 Example 2: Workforce Planning in Maintenance 360
13.4.1 Problem Description 360
13.4.2 Procedure 361
13.4.3 Case Study and Results 362
13.4.4 Summary 363
13.5 Example 3: A Simple Case Performed by Hand Calculations 363
13.5.1 Case Description 363
13.5.2 Study Conditions and Data 365
13.5.3 EENS Evaluation 365
13.5.4 Summary 367
13.6 Conclusions 367
14 Probabilistic Spare-Equipment Analysis 369
14.1 Introduction 369
14.2 Spare-Equipment Analysis Based on Reliability Criteria 370
14.2.1 Unavailability of Components 370
14.2.2 Group Reliability and Spare-Equipment Analysis 372
14.3 Spare-Equipment Analysis Using the Probabilistic Cost Method 373
14.3.1 Failure Cost Model 373
14.3.2 Unit Failure Cost Estimation 374
14.3.3 Annual Investment Cost Model 375
14.3.4 Present Value Approach 375
14.3.5 Procedure of Spare-Equipment Analysis 376
14.4 Example 1: Determining Number and Timing of Spare Transformers 376
14.4.1 Transformer Group and Data 376
14.4.2 Spare-Transformer Analysis Based on Group Failure Probability 377
14.4.3 Spare-Transformer Plans Based on the Probabilistic Cost Model 378
14.4.4 Summary 381
14.5 Example 2: Determining Redundancy Level of 500 kV Reactors 381
14.5.1 Problem Description 381
14.5.2 Study Conditions and Data 383
14.5.3 Redundancy Analysis 385
14.5.4 Summary 387
14.6 Conclusions 387
15 Asset Management Based on Condition Monitoring and Risk Evaluation 389
15.1 Introduction 389
15.2 Maintenance Strategy of Overhead Lines 390
15.2.1 Risk Evaluation Using Condition Monitoring Data 391
15.2.2 Overhead Line Maintenance Strategy 397
15.2.3 Case Study 399
15.2.4 Summary 401
15.3 Replacement Strategy for Aged Transformers 402
15.3.1 Transformer Aging Failure Unavailability Using Condition Monitoring Data 403
15.3.2 Transformer Replacement Strategy 407
15.3.3 Case Study 410
15.3.4 Summary 413
15.4 Conclusions 414
16 Reliability-Based Transmission-Service Pricing 417
16.1 Introduction 417
16.2 Basic Concept 418
16.2.1 Incremental Reliability Value 419
16.2.2 Impacts of Customers on System Reliability 420
16.2.3 Reliability Component in Price Design 421
16.3 Calculation Methods 422
16.3.1 Unit Incremental Reliability Value 422
16.3.2 Generation Credit for Reliability Improvement 423
16.3.3 Load Charge for Reliability Degradation 423
16.3.4 Load Charge Rate Due to Generation Credit 424
16.4 Rate Design 424
16.4.1 Charge Rate for Wheeling Customers 424
16.4.2 Charge Rate for Native Customers 425
16.4.3 Credit to Generation Customers 425
16.5 Application Example 425
16.5.1 Calculation of the UIRV 427
16.5.2 Calculation of the GCRI 427
16.5.3 Calculation of the LCRD 427
16.5.4 Calculation of the LCRGC 428
16.5.5 Calculations of Charge Rates 428
16.6 Conclusions 430
17 Voltage Instability Risk Assessment and its Application to System Planning 431
17.1 Introduction 431
17.2 Method of Assessing Voltage Instability Risk 432
17.2.1 Maximum Loadability Model for System States 432
17.2.2 Models for Identifying Weak Branches and Buses 436
17.2.3 Determination of Contingency System States 443
17.2.4 Procedure of Calculating Voltage Instability Risk Indices 444
17.3 Tracing and Locating Voltage Instability Risk for Planning Alternatives 447
17.4 Case Studies 448
17.4.1 Results of the IEEE 14-Bus System 448
17.4.2 Results of the 171-Bus Utility System 453
17.5 Conclusions 456
18 Probabilistic Transient Stability Assessment 459
18.1 Introduction 459
18.2 Probabilistic Modeling and Simulation Methods 460
18.2.1 Selection of Pre-Fault System States 460
18.2.2 Fault Models 461
18.2.3 Monte Carlo Simulation of Fault Events 463
18.2.4 Transient Stability Simulation 464
18.3 Procedure 464
18.3.1 Procedure for the First Type of Study 465
18.3.2 Procedure for the Second Type of Study 465
18.4 Examples 465
18.4.1 System Description and Data 465
18.4.2 Transfer Limit Calculation in the Columbia River System 470
18.4.3 Generation Rejection Requirement in the Peace River System 472
18.4.4 Summary 475
18.5 Conclusions 475
Appendix A Basic Probability Concepts 477
A.1 Probability Calculation Rules 477
A.1.1 Intersection 477
A.1.2 Union 477
A.1.3 Full Conditional Probability 478
A.2 Random Variable and its Distribution 478
A.3 Important Distributions in Risk Evaluation 479
A.3.1 Exponential Distribution 479
A.3.2 Normal Distribution 479
A.3.3 Log-Normal Distribution 481
A.3.4 Weibull Distribution 481
A.3.5 Gamma Distribution 482
A.3.6 Beta Distribution 483
A.4 Numerical Characteristics 483
A.4.1 Mathematical Expectation 483
A.4.2 Variance and Standard Deviation 484
A.4.3 Covariance and Correlation Coefficients 484
A.5 Nonparametric Kernel Density Estimator 485
A.5.1 Basic Concept 485
A.5.2 Determination of the Bandwidth 486
Appendix B Elements of Monte Carlo Simulation 489
B.1 General Concept 489
B.2 Random Number Generators 490
B.2.1 Multiplicative Congruent Generator 490
B.2.2 Mixed Congruent Generator 491
B.3 Inverse Transform Method of Generating Random Variates 491
B.4 Important Random Variates in Risk Evaluation 492
B.4.1 Exponential Distribution Random Variate 492
B.4.2 Normal Distribution Random Variate 493
B.4.3 Log-Normal Distribution Random Variate 494
B.4.4 Weibull Distribution Random Variate 494
B.4.5 Gamma Distribution Random Variate 495
B.4.6 Beta Distribution Random Variate 495
Appendix C Power Flow Models 497
C.1 AC Power Flow Models 497
C.1.1 Power Flow Equations 497
C.1.2 Newton–Raphson Method 497
C.1.3 Fast Decoupled Method 498
C.2 DC Power Flow Models 499
C.2.1 Basic Equation 499
C.2.2 Line Flow Equation 500
Appendix D Optimization Algorithms 503
D.1 Simplex Methods for Linear Programming 503
D.1.1 Primal Simplex Method 503
D.1.2 Dual Simplex Method 505
D.2 Interior Point Method for Nonlinear Programming 506
D.2.1 Optimality and Feasibility Conditions 506
D.2.2 Procedure of the Algorithm 508
Appendix E Three Probability Distribution Tables 511
References 515
Further Reading 523
Index 525