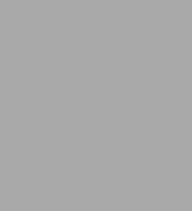
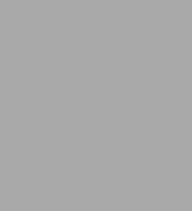
Paperback(SPIRAL)
-
SHIP THIS ITEMNot Eligible for Free ShippingPICK UP IN STORECheck Availability at Nearby Stores
Available within 2 business hours
Related collections and offers
Overview
Product Details
ISBN-13: | 9780854046010 |
---|---|
Publisher: | RSC |
Publication date: | 01/03/2000 |
Edition description: | SPIRAL |
Pages: | 270 |
Product dimensions: | 8.25(w) x 11.70(h) x (d) |
Read an Excerpt
Interpreting Organic Spectra
By David Whittaker
The Royal Society of Chemistry
Copyright © 2000 The Royal Society of ChemistryAll rights reserved.
ISBN: 978-0-85404-601-0
CHAPTER 1
Infrared Spectroscopy
Infrared spectroscopy is the best means of identifying functional groups in a molecule. It involves measuring the absorption by a substance of radiation in the region from 4000 to 600 cm-1. The range covers roughly 2.5–16 µm (1 µm = 10-4 cm), but the frequency scale is now used universally.
Every bond in a molecule vibrates, resulting in a change in its dipole moment. This change in dipole moment provides a mechanism for the absorption of radiation. The energy of the vibration is such that the radiation absorbed is in the infrared region, which is of lower frequency, and hence lower energy, than visible light. Consequently, every bond in a molecule has an absorption peak in the infrared spectrum or the Raman spectrum of the molecule. Every substance therefore has its own unique infrared spectrum, so that we can identify any organic material by comparing its infrared spectrum with that of a known sample. In addition, each different functional group, such as O–H, C–H or C=C, absorbs within a narrow range of frequencies so that we can identify a functional group present in a molecule by the presence of an absorption band in a particular range of the infrared spectrum.
The frequency at which a bond absorbs radiation depends on the masses of the atoms forming the bond. The bonds which absorb radiation at the upper end of the frequency range are those which involve a light atom, hydrogen, with a heavier atom, such as nitrogen, oxygen or carbon. Hydrogen, being light, vibrates strongly and rapidly, so we see a strong, high energy absorption. As we move to lower frequencies, we come to the vibrations of two heavier atoms, such as C–N, C–O and C–C, and finally, at the lowest energies, we find the vibrations of the heavier atoms bonded to very heavy atoms, such as C–Cl, C–Br and C–I. Within this general trend, multiple bonds absorb higher energy radiation than single bonds, so the C [equivalent to] C bond absorbs at higher frequency than the C=C bond.
Before we study an infrared spectrum, we should consider how it is obtained. The sample to be studied is usually examined in one of four ways:
(1) As a pure liquid.
(2) As a mull. This method is used for solids. The solid is ground finely using a pestle and mortar, and mixed with a small amount of a liquid hydrocarbon (liquid paraffin) and run as a liquid. This has the disadvantage that the spectrum of the liquid hydrocarbon is super imposed on the spectrum of the sample.
(3) The compound is finely ground, mixed with K Br, compressed and run as a disc. This avoids adding extra absorption, but is time consuming.
(4) The compound is dissolved in a suitable solvent, and run as a solution. The spectrum cannot be observed in regions where the solvent absorbs.
Now let us consider an infrared spectrum. The spectrum of phenylamine, PhNH2 (also known as aniline), is shown in Figure 1.1.
The spectrum records the amount of radiation transmitted at each frequency, so the maximum absorption occurs when the least light is transmitted, and the recorder line is closest to the bottom of the spectrum. Positions of maximum absorption are difficult to measure accurately from the spectrum, so most spectrometers record them automatically, and print them alongside the spectrum, giving the % of radiation transmitted (%T) alongside. The machine can be set to record frequencies of all or only the stronger peaks.
Phenylamine has an -NH2 group, which characteristically absorbs in the region from 3500 to 3250 cm-1. The spectrum actually has two peaks in this range, at 3426 and 3352 cm-1. These do NOT represent a peak for each N-H bond (the bonds are indistinguishable so must have identical absorption frequencies): they result from the in-phase and out-of-phase vibrations of the N–H bonds:
[FORMULA NOT REPRODUCIBLE IN ASCII]
If the N–H bond is part of an amide, hydrogen bonding moves the absorption to lower frequency. If we have a secondary amine, with only one N–H bond, we obtain a single peak, which can easily be confused with the O–H bond.
A genuine O-H bond is shown in the next spectrum, that of butan-2-0l, CH3CH2CH(OH)CH3. This is shown in Figure 1.2. The O–H bond absorbs radiation in the range 3700–3200 cm-1, and we find that butan-2-01 has a peak at 3338 cm'. The O–H peak, like the N–H peak but unlike other peaks in the spectrum, is a broad rounded peak rather than the usual sharp spiked peaks. This is a result of intermolecular hydrogen bonding. The hydrogen atom on any particular oxygen atom is probably attached to another oxygen atom by hydrogen bonding, so bond vibrations vary over a frequency range, and the broad peak which we see is an envelope covering many absorptions at slightly different frequencies. If we dilute the sample of butan-2-01 with solvent which cannot form a hydrogen bond, such as dichloromethane, we get the spectrum shown in Figure 1.3, in which the broad O–H bond peak is reduced, and a small sharp absorption peak has appeared at higher frequency, resulting from the non-hydrogen bonded O–H bond. At greater dilution of the alcohol dissolved in dichloromethane, the original O–H peak has almost vanished, and the non-hydrogen bonded peak has further increased in size, as shown in Figure 1.4.
The O–H bond of a carboxylic acid shows even stronger hydrogen bonding than that of an alcohol, since the acid is so strongly hydrogen bonded as to exist in the dimeric form in the pure liquid:
[FORMULA NOT REPRODUCIBLE IN ASCII]
As a result, the carboxylic acid O–H group has an extremely broad absorption peak in the range 3200–2200 cm-1. Combined with a carbonyl peak in the range 1725–1680 cm-1, this makes identification of a carboxylic acid from its infrared spectrum an easy task. A typical carboxylic acid spectrum is that of benzoic acid, PhCOOH, which is shown in Figure 1.5.
As we move to lower frequencies in the infrared range, the next peak that we encounter is the C–H peak in the region 3100–2700 cm-1. This supplies little useful structural information since almost all organic compounds contain hydrogen, and in the case of a solid run as a mull it will certainly be present in the liquid hydrocarbon used to make the mull. After the C–H absorption, we leave the high-frequency high-intensity X–H bonds, and, after a usually blank region of the spectrum, encounter the weaker triple bond absorption frequencies. The most common are the C [equivalent to] N bond, which absorbs at 2300–2200 cm-1, and the C [equivalent to] C bond, which absorbs in the region from 2250 to 2100 cm-1. This latter bond is particularly weak if neither carbon atom is bonded to hydrogen. The carbonyl group occurs next, absorbing over the unusually wide range of 1850–1640 cm-1. However, the variation of the position of the C=O peak within this range is very valuable diagnostically, and will be considered separately.
As we further descend the frequency scale, we next come to the C=C bond region, which covers 1680–1620cm-1 in unconjugated systems, and can go as low as 1590 cm-1 in conjugated systems. The C=C bond is often of low intensity, especially when fully substituted, and it is easily missed. In an aromatic system, the C–C bonds usually have two or three absorption peaks between approximately 1600 and 1500 cm-1, usually with the strongest peak at about 1500 cm-1. The spectrum of benzoic acid shown in Figure 1.5 illustrates this with weak peaks at 1601 cm-1 and 1583cm-1, and a stronger peak at 1496 cm.
The region below about 1500cm-1 is usually complex, and contains relatively little information about functional groups. It is often referred to as the 'fingerprint region', since this is the region of greatest value when comparing the spectrum of an unknown compound with that of a known compound. At the lower frequency end of this region we come to the C–C1 bond absorption in the region 800–400 cm-1. The C–Br and C–I bonds usually absorb at too low a frequency for most spectrometers. The halogens are more readily identified by mass spectrometry.
The absorbing frequencies discussed above have been collected together in Table 1.1.
The carbonyl absorption was referred to earlier as covering a very wide range of frequencies. The absorption frequency is unusually sensitive to substitution at the carbonyl carbon atom. The reason for this is that the carbonyl group exists as a resonance hybrid of two forms:
C=O [left and right arrow] C+-O-
The two forms absorb at different frequencies, the polar C+-O- having the higher frequency absorption. If we now consider the hybrid
[FORMULA NOT REPRODUCIBLE IN ASCII]
it is easy to see that electron withdrawing substituents will favour the C+-O- form, and electron supplying substituents will favour the C=O form.
When the substituent X is a chlorine atom, this strongly electron withdrawing group favours the C+-O- bond, so acid chlorides absorb in the region around 1815–1790cm-1. The carbonyl group is another electron withdrawing substituent, so that the anhydride absorbs in this region, but since we have two carbonyl groups close together we can have in-phase and out-of-phase vibrations, as with the NH2 group. Saturated carboxylic acid anhydrides thus show two peaks, one in the region 1850–1800 cm-1 and the other in the region 1790–1740 cm-1.
The position of the carbonyl peak, however, does not depend entirely on inductive effects. In esters, the overlap of the lone pair of electrons on X with the C=O bond reduces the double bond character of the C=O bond, so the ester carbonyl group absorbs in the range 1755–1735 cm-1. Aldehydes and ketones come next at 1740–1700 cm-1, then the carboxylic acid carbonyl absorbs at 1725-1700 cm-1, which is lower than might have been expected, but its position is affected by the dimeric nature of the carboxylic acid group in solution.
All these examples have referred to the carbonyl carbon atom attached to a saturated group; if it is attached to a multiple bond or an aromatic ring, then the carbonyl absorption frequency is lowered by 15–40 cm-1. Thus, if we need to assign a carbonyl group, it is first necessary to see if the molecule shows signs of unsaturation; if this is next to the carbonyl carbon atom, then carbonyl frequencies will be lower than expected. Unexpectedly, conjugation shifts the amide carbonyl to higher frequency. It may be due to an inductive effect on the conjugated CO–N system.
The carbonyl absorption frequencies have been collected together in Table 1.2.
Problems in Interpreting Infrared Spectra
Using the data given in Tables 1.1 and 1.2, you should now be able to identify the common functional groups present in the compounds whose spectra comprise Samples 1 to 20. Remember that it is not sufficient to identify a compound as simply a carbonyl compound. If you observe a carbonyl peak, you should check for the presence of an aromatic ring or a double bond in the molecule, then use the data in Table 1.2 to say what type of carbonyl group (e.g. anhydride, ketone) is present in the sample. Note that some spectra are consistent with more than one structural type, since ranges of frequencies can overlap. All the spectra are run on pure liquids or nujol mulls.
CHAPTER 2Mass Spectrometry
Mass spectrometry gives us some information about the structure of a molecule. It can usually tell us the molecular weight of a substance, and can detect the presence of bromine, chlorine and iodine. It may be possible to deduce the structure of a compound from its mass spectrum.
Unlike the other techniques studied in this book, it is a spectrometric, not a spectroscopic, method. It does not involve the absorption of radiation; it consists of generating ions of such energy that they fragment, collecting fragments of the same mass together, and weighing them. Some fragments are more stable than others, and so are produced to a much greater extend than others, and we see large peaks in the spectrum at their mass. Using knowledge of favoured fragmentations, a surprising amount of information about the structure of the original molecule can be obtained.
Mass spectrometers operate at very low pressures, so that the ions can travel long distances without undergoing collisions. The mass spectrometer consists basically of three parts, as shown schematically in Diagram 2.1. The regions of the spectrometer can be described according to their function, as ionisation, separation and collection.
Ionisation
The material to be studied is introduced into the ionisation chamber in the vapour phase. It is bombarded with a stream of electrons, generated by the filament and accelerated to about 70 eV by the anode.
If an electron at this energy hits one of the atoms being studied, the atom gains considerable vibrational energy, causing it to ionise and usually fragment. The ions produced pass through a series of slits into the analysing system.
This method of ionisation is used for most samples which are reasonably stable and reasonably volatile. A number of other ionisation methods exist to tackle less stable or less volatile samples, but all work on the same principle: the molecule absorbs energy and breaks down to its more stable ions.
Ion separation
Ionisation of a compound gives a series of ions of different masses, but almost all carry a single positive charge. The stream of positive ions needs to be separated according to the mass to charge ratio, usually denoted by m/z, which effectively separates them by mass, as z = 1. This is done by passing the beam through a magnetic field, which deflects lighter ions more than heavier ions. The actual amount of deflection is dependent on the mass to charge ratio, m/z. At the end of the travel through the magnetic field, the beam passes through a narrow slit, and proceeds to the collector. By varying the magnetic field, ions of any m/z ratio can be focused on the collector slit, and the spectrum scanned.
Most mass spectrometers use a more complex arrangement of electrostatic and magnetic analysers in order to improve the resolution of the instrument, but the principle involved is the same.
Ion collection
When the ions reach the collector, ions of each m/z ratio land on the collector plate for a similar interval of time, and build up a charge which is amplified and recorded.
One of the great advantages of mass spectrometry is that it can work with extremely small samples, less than 10-12 of a mole. It is often used coupled to a gas–liquid chromatography analyser, and the separated fractions are run automatically into the mass spectrometer. It is particularly valuable for the study of biological samples.
The mass spectrometer ionises the molecules, then divides the ions up according to their m/z ratio, and measures the amount of ions of each m/z ratio. The result is presented as a bar chart, as shown in Figure 2.1, the mass spectrum of n-octane, CH3CH2CH2CH2CH2 CH2CH2CH3.
Along the base of the chart, the scale is in m/z ratio values, but since z = 1, these can be regarded as the masses of the ions. The vertical scale shows the amount of each ion. Since the amount of any ion collected obviously depends on how long the collector plate has been in the ion beam, we adopt an arbitrary scale for intensity, in which the largest peak is given an intensity value of 100 and is known as the parent peak. All other peaks have their intensities measured as a percentage of the parent peak.
Before we start to look at the structural information which can be obtained, we need to consider the information which can be obtained from isotope masses and ratios. One of the most useful things we can do is to measure the accurate mass of a peak. The atomic weights of most isotopes are not quite integral numbers, and most have been measured accurately to six places of decimals. A few useful accurate masses are given in Table 2.1.
(Continues...)
Excerpted from Interpreting Organic Spectra by David Whittaker. Copyright © 2000 The Royal Society of Chemistry. Excerpted by permission of The Royal Society of Chemistry.
All rights reserved. No part of this excerpt may be reproduced or reprinted without permission in writing from the publisher.
Excerpts are provided by Dial-A-Book Inc. solely for the personal use of visitors to this web site.