Coordination Polymers: Design, Analysis and Application / Edition 1 available in Hardcover
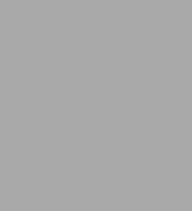
Coordination Polymers: Design, Analysis and Application / Edition 1
- ISBN-10:
- 0854048375
- ISBN-13:
- 9780854048373
- Pub. Date:
- 11/06/2008
- Publisher:
- RSC
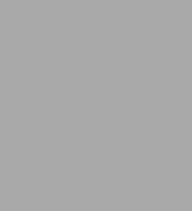
Coordination Polymers: Design, Analysis and Application / Edition 1
Hardcover
Buy New
$196.00Buy Used
$144.72-
-
SHIP THIS ITEM
Temporarily Out of Stock Online
Please check back later for updated availability.
-
Overview
Product Details
ISBN-13: | 9780854048373 |
---|---|
Publisher: | RSC |
Publication date: | 11/06/2008 |
Pages: | 424 |
Product dimensions: | 6.30(w) x 9.30(h) x 1.10(d) |
About the Author
Read an Excerpt
Coordination Polymers
Design, Analysis and Application
By Stuart R. Batten, Suzanne M. Neville, David R. Turner
The Royal Society of Chemistry
Copyright © 2009 Stuart R. Batten, Suzanne M. Neville and David R. TurnerAll rights reserved.
ISBN: 978-0-85404-837-3
CHAPTER 1
Introduction
1.1 Introduction
Sometime between 1704 and 1705, a Berlin colourmaker named Diesbach made a mistake. He was trying to make a red pigment known as cochineal red lake. The recipe was simple – iron sulfate and potash. But it turned out pale. Upon further concentration, it became deep blue! By using cheap potash, contaminated with animal oil made from ox blood, Diesbach had created Prussian Blue. This was the first man-made coordination polymer and in fact the first man-made coordination compound. It was also a valuable pigment; within a few short years it was being made commercially from a closely guarded recipe.
It would, however, be another 372 years before the structure of Prussian Blue, Fe4[Fe(CN)6]3·x H2O, would be determined (Figure 1.1a). In the intervening years, little attention was paid to coordination polymers (certainly much less than their organic cousins received), with only a few scattered structural studies. The structures of Zn(CN)2 and Cd(CN)2 were reported by a Russian group in the depths of World War II. Powell and Rayner determined the structures of the Hofmann clathrate, [Ni(NH3)2Ni(CN) 4]·2C6H6, shortly afterwards (Figure 1.1b); this work was later extensively followed up by Iwamoto's group on related compounds. In 1959, a Japanese group reported, remarkably, that they had determined that the structure of [Cu(adiponi-trile)]NO3 contained six interpenetrating diamond networks. The ID chain structures of Ag(pyrazine)NO3 and Cu(pyrazine)(NO3)2 were reported in 1966 and 1970, respectively. The crystal structure of Co(pyrazine)2 Cl2 was shown to have a square grid structure in 1971.
As the 1980s came to a close, there was increasing interest in these materials, particularly in the field of molecule-based magnetic materials. However, it was not until a short communication in 1989, and a subsequent full paper in 1990, that interest really took off.
In these and subsequent papers, Robson, Hoskins and co-workers outlined a net-based approach to the design of coordination polymers. They took the landmark work of Wells, which described crystal structures in terms of networks, and applied it to the design of new coordination polymers (Figure 1.2). Through this design approach, they proposed that new materials with interesting properties such as porosity and catalysis could be deliberately engineered. These ideas soon caught on, with other early groups in the field making important contributions that would ultimately lead to the explosion in research illustrated in Figure 1.3.
1.2 Crystal Engineering, Supramolecular Chemistry, Metallosupramolecules
The development of coordination polymer research was reinforced by the growth of two other closely related areas: crystal engineering and supramolecular chemistry (particularly metallosupramolecular chemistry).
Crystal engineering seeks to understand why molecules pack in the ways that they do and to use that knowledge to deliberately engineer the arrangements of molecules in new materials. This is important because the properties of materials are often governed by the way in which their constituent molecules are arranged. Control over this arrangement gives control over the properties.
In 'molecular' (largely organic) crystal engineering, the interactions are weaker than coordination bonds and can range in strength from very strong hydrogen bonding to weak C–H ··· A hydrogen bonds, halogen bonds, π interactions and, ultimately, van der Waals forces. The crystal engineer seeks to understand and harness all these interactions. However, despite the differences in the interactions, there is much that is common in these two areas. Indeed, coordination polymers, which essentially exist only in the solid state, should be considered as a subset of crystal engineering. Furthermore, the net-based approach for coordination polymers is equally valid for molecular species connected by well-defined interactions. For example, trimesic acid (benzene-1,3, 5-tricarboxylic acid) readily forms hexagonal sheets in which the molecules are connected by hydrogen bonding, as shown in Figure 1.4a. The large organic molecule shown in Figure 1.4b assembles, as one would predict, into seven interpenetrating diamond networks through hydrogen bonding between the peripheral functional groups.
Many of the concepts and terminology in molecular crystal engineering also apply to coordination polymers. Interactions between molecules that direct their packing arrangements (such as the hydrogen bonding carboxylate dimer motif in Figure 1.4a) are known as supramolecular synthons; in coordination polymers, the main synthons are coordination bonds (although weaker synthons can also be important, as discussed in Chapter 4). The building blocks used to create the structure, such as the molecules shown in Figure 1.4, are called tectons; for coordination polymers, the tectons are metal ions and ligands. These two concepts are highlighted in Figure 1.5.
The aim of supramolecular chemistry is similar: to create assemblies of molecules, that is, not to create structures an atom at a time, but to design molecules such that when combined they spontaneously self-assemble in a predetermined fashion into larger architectures. Thus crystal engineering can, in fact, be considered to be the supramolecular chemistry of the solid state. To quote Dunitz, 'The crystal is, in a sense, the supramolecule par excellence ...'.
The supramolecular chemist, like the crystal engineer, uses a range non-covalent intermolecular interactions, including hydrogen bonding and coordination bonds. Use of the later gives rise to metallosupramolecular chemistry, and much of the design and indeed the structures obtained have close relationships to coordination polymers. For example, the design and chemistry may be similar, except that the use of a convergent ligand building block will give a metallosupramolecule whereas a divergent one will generate a polymer (Figure 1.6a). Alternatively, even the same bridging ligand can be used, with construction of a metallosupramolecule being directed by the use of 'capping' chelating co-ligands on the metals [such as 2,2'-bipyridine, ethy-lenediamine (en), 1,10-phenanthroline, 1,4,7-triazacyclononane (TACN), cyclopentadiene]. In the absence of these capping groups, polymers are formed (Figure 1.6b). Despite the different products, both areas have the same modular approach to the design and synthesis and similar (or even the same) building blocks.
Even the architectures achieved in the two fields can be similar. The supra-molecule shown in Figure 1.7a, constructed from 4, 4'-bipyridine (4,4'-bipy) and (en)PdII, has the same structure as the windows in the 2D coordination polymer obtained from reaction of the same ligand with ZnSiF6 (Figure 1.7b).The molecular cube in Figure 1.7c has the same connectivity as the cavities in Prussian Blue. Reaction of 2,4,6-tri(4-pyridyl)-1,3,5-triazine (tpt) with (en) PdII gives the discrete cages shown in Figure 1.7d; a similar reaction with CuI generates a 3D polymer which contains the same cages (Figure 1.7e). In the later structure there are no capping groups on the metal to terminate the structure and thus a polymer is generated in which the cages are connected by shared metal atoms.
1.3 What is a Coordination Polymer?
A coordination polymer contains metal ions linked by coordinated ligands into an infinite array. This infinite net must be denned by coordination bonds and thus molecular species linked only by hydrogen bonding, such as the example shown in Figure 1.8, are elegant instances of molecular crystal engineering but are not coordination polymers. Similarly, a structure linked by coordination bonds in one direction and hydrogen bonds it two other directions is a ID coordination polymer (although an overall 3D net may be defined by both sets of interactions).
We also exclude here more 'inorganic' materials, such as halides, oxides, hydroxides, alkoxides, sulfides and polyoxides (sulfates, phosphates, etc.,, although we do include pseudohalides such as cyanide, azide and thiocyanate. Furthermore, for the purposes of this book, we largely ignore alkali and alkaline earth metals, which have more ionic bonding, and main group metals, in which the bonding is more covalent, and thus focus largely on the transition and lanthanoid ions.
There are good reasons for doing this. One feature of the design of coordination polymers is that the strength and lability of the coordination bond are such that ordered materials can be readily synthesised because of the reversibility of these interactions. Unlike covalently bonded organic polymers, in which the bonds are largely irreversible, errors in the assembly of a coordination polymer can be readily corrected during growth so that a periodic 3D structure with crystallographic order can be achieved. By contrast, in an organic polymer mistakes are 'locked in' once made, resulting in a material with much less periodic ordering. This ordering of coordination polymers allows detailed structural determination through X-ray crystallography and, through this, precise structure–property correlations. It is also important to the properties themselves, e.g. a regularity and consistency of pore size and environment that cannot be achieved with amorphous materials. On the other hand, the coordination bond is also strong enough to provide robust materials and good electronic and magnetic communication between metal centres. It is also directional, with generally predictable geometries around the metal centre (particularly for transition metals), allowing design to be attempted with some degree of confidence.
A coordination polymer thus consists essentially of metal and ligands, although they often include guests and counterions. The metal ions, as discussed above, are usually transition metals and/or lanthanoids. For transition metals, the field is dominated by the first-row elements (plus Zn, Cd, Hg, Ag and, to a lesser extent, Au, Pd, Pt), due to their kinetic lability and ready availability and stability. Generally, transition metals have been more popular, due in part to the more predictable nature of their coordination geometries; however, lanthanoids have attracted increased attention recently, with their higher connectivity leading to interesting topologies, in addition to other inherent properties of interest (e.g. luminescence). These metals are commonly used as their halide, nitrate, perchlorate, tetrafluoroborate, hexafluorophosphate, hexafluorosilicate or triflate salts; 'non-coordinating' anions are usually preferred.
Although the nature of metal salt chosen is important, the real variation in coordination polymers comes through the infinite variability and creativity of ligand design. Nonetheless, there are a number of features that are common to most ligands used (Figure 1.9). Typically, a ligand might have two divergent coordination sites (although there are many examples of higher connectivity), and these coordination sites are usually pyridyl, imidazole, nitrile or carboxylate functional groups (discounting the large body of pseudohalide work). These ligands can also range from the very rigid to the completely flexible, with the corresponding loss in predictability.
1.4 Synthetic Techniques
One of the challenges of this research is to obtain single crystals suitable for detailed crystallographic analysis. Unlike molecular species, most coordination polymers are insoluble once synthesised (a property which is advantageous for other aspects) and so recrystallisation is not an option. If the polymers can be dissolved, it is usually through the use of strongly coordinating solvents, which are then likely to become part of the recrystallised species, which therefore becomes a different material to the original phase.
Crystals are therefore usually obtained directly from the synthetic reaction mixtures. Although some species crystallise nicely from directly mixed solutions, for other systems the key to obtaining good crystals is to slow the precipitation down. This is most commonly done by allowing two separate solutions of metals and ligands to diffuse slowly into each other, and a number of different techniques have been established to this end (Figure 1.10). The simplest method is to layer carefully one solution on top of another in a small vial or tube. Often a buffer layer of pure solvent is layered between the two and the use of solvents with different densities (e.g. MeOH versus CHCI3) greatly aids separation. This layered solution should then be left so that the crystals can grow; typically this may take in the order of 2 weeks, although crystallisation can often take much longer (or shorter) times and so the reaction should be checked regularly, preferably without disturbing the crystal growth through handling. Regular inspection is important as crystals can come and go (for kinetic products) or become flawed, overgrown or otherwise deteriorate in quality over time.
Other variations on this technique include locking one solution into a gel through the addition of a gelling agent such as tetramethoxysilane. The gel slows diffusion through reduction of convection and also provides a support for the growing crystals. Specially designed glassware such as H-tubes and U-tubes (Figure 1.10) can also be used; often these can have a frit in the middle or (in the case of U-tubes) a separating gel plug can be created at the bottom first.
As detailed in Chapter 4, there are a number of factors that contribute to stable crystalline packing arrangements. For the synthetic chemist, this means that there are therefore a number of other variables that can be adjusted to produce crystals. Variation of solvent, counterion or even metal choice can be explored, as can synthetic tweaks to the ligands. More recently, the use of solvothermal techniques has become increasingly popular, both as a method of obtaining good single crystals and as a means of obtaining phases which are unavailable through bench-top techniques.
There is, overall, a large parameter space which can be explored in the quest for single crystals. However, one of the key reasons for obtaining crystal structures is to draw relationships between structures and properties and thus gain insights that can feed into the design of new materials. Therefore, it is important to recognise that the structures obtained from single crystals may be inherently unrepresentative (because the crystallographer chooses the best crystal available, for obvious reasons) of the bulk material upon which the properties are tested. Furthermore, reactions can often give more than one product. Hence it is important to check the correlation between the single crystals and the bulk product, and this is most easily achieved through the use of techniques such as powder diffraction or (less convincingly) infrared or Raman spectroscopy.
Finally, although we largely focus in this book on materials characterised through single-crystal crystallography, the structures of some simple coordination polymers have been determined directly through powder diffraction. Powder diffraction can also be used to correlated known structures and new microcrystalline materials. These latter materials may be analogous to known structures but lacking in single crystals (e.g. the same structure but different metals) or synthesised using unusual techniques, such as solid-state decomposition or mechanochemical techniques. Furthermore, non-crystalline materials can also have very interesting properties even if the detailed structure is unknown. The compound V(tcne)2·-0.5CH2Cl2, for example, has shown magnetic ordering above room temperature. This material has unfortunately only ever been obtained as an amorphous powder, so its structure, which is no doubt key to its magnetism, remains unknown. But it is still a magnet.
1.5 Design, Analysis, Application
The rest of this book broadly follows the themes of design, analysis and application. Chapter 2 deals with design (nets), Chapter 3 deals with some of the consequences of nets (interpenetration) and Chapter 4 examines in detail the many other aspects that should be taken into account when designing or examining a structure. In the following four chapters, we provide an extensive analysis of reported coordination polymers and related areas such as organo-metallic networks and inorganic–organic hybrid materials. Finally, we look at the application of these materials to a number of fields, including magnetism (long-range ordering, spin crossover), porosity (gas storage, ion and guest exchange), non-linear optical activity, chiral networks, reactive networks, heterogeneous catalysis, luminescence, multifunctional materials and other assorted properties. The examples given throughout have been chosen based on their ability to illustrate a point, their historical significance or the fact that they are either typical or atypical (even exceptional) of a larger class of materials. In many cases, the choice is purely subjective and readers are directed to a long list of literature reviews available as additional complementary resources.
CHAPTER 2Nets: A Tool for Description and Design
2.1 Introduction
One of the most powerful techniques in crystal engineering for both the analysis and design of solids is to reduce their crystal structures to networks (or nets). Networks can aid the description and understanding of complicated structures or provide a blueprint for the targeting of particular packing arrangements and their associated properties.
An early leading figure in this approach was A. F. Wells, who, in a series of seminal books, described a number of molecular and polymeric structures in terms of networks and delineated a large number of possible networks, some already seen in real structures and others that, remarkably, were still theoretical at the time.
(Continues...)
Excerpted from Coordination Polymers by Stuart R. Batten, Suzanne M. Neville, David R. Turner. Copyright © 2009 Stuart R. Batten, Suzanne M. Neville and David R. Turner. Excerpted by permission of The Royal Society of Chemistry.
All rights reserved. No part of this excerpt may be reproduced or reprinted without permission in writing from the publisher.
Excerpts are provided by Dial-A-Book Inc. solely for the personal use of visitors to this web site.