Two leading physicists discuss the importance of the Higgs Boson, the future of particle physics, and the mysteries of the universe yet to be unraveled. On July 4, 2012, the long-sought Higgs Boson--aka "the God Particle"--was discovered at the world's largest particle accelerator, the LHC, in Geneva, Switzerland. On March 14, 2013, physicists at CERN confirmed it. This elusive subatomic particle forms a field that permeates the entire universe, creating the masses of the elementary particles that are the basic building blocks of everything in the known world--from viruses to elephants, from atoms to quasars. Starting where Nobel Laureate Leon Lederman's bestseller The God Particle left off, this incisive new book explains what's next. Lederman and Hill discuss key questions that will occupy physicists for years to come:* Why were scientists convinced that something like the "God Particle" had to exist?* What new particles, forces, and laws of physics lie beyond the "God Particle"?* What powerful new accelerators are now needed for the US to recapture a leadership role in science and to reach "beyond the God Particle," such as Fermilab's planned Project-X and the Muon Collider? Using thoughtful, witty, everyday language, the authors show how all of these intriguing questions are leading scientists ever deeper into the fabric of nature. Readers of The God Particle will not want to miss this important sequel.
Two leading physicists discuss the importance of the Higgs Boson, the future of particle physics, and the mysteries of the universe yet to be unraveled. On July 4, 2012, the long-sought Higgs Boson--aka "the God Particle"--was discovered at the world's largest particle accelerator, the LHC, in Geneva, Switzerland. On March 14, 2013, physicists at CERN confirmed it. This elusive subatomic particle forms a field that permeates the entire universe, creating the masses of the elementary particles that are the basic building blocks of everything in the known world--from viruses to elephants, from atoms to quasars. Starting where Nobel Laureate Leon Lederman's bestseller The God Particle left off, this incisive new book explains what's next. Lederman and Hill discuss key questions that will occupy physicists for years to come:* Why were scientists convinced that something like the "God Particle" had to exist?* What new particles, forces, and laws of physics lie beyond the "God Particle"?* What powerful new accelerators are now needed for the US to recapture a leadership role in science and to reach "beyond the God Particle," such as Fermilab's planned Project-X and the Muon Collider? Using thoughtful, witty, everyday language, the authors show how all of these intriguing questions are leading scientists ever deeper into the fabric of nature. Readers of The God Particle will not want to miss this important sequel.
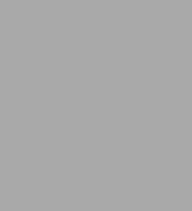
Beyond the God Particle
336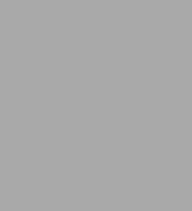
Beyond the God Particle
336eBook
Available on Compatible NOOK devices, the free NOOK App and in My Digital Library.
Related collections and offers
Overview
Two leading physicists discuss the importance of the Higgs Boson, the future of particle physics, and the mysteries of the universe yet to be unraveled. On July 4, 2012, the long-sought Higgs Boson--aka "the God Particle"--was discovered at the world's largest particle accelerator, the LHC, in Geneva, Switzerland. On March 14, 2013, physicists at CERN confirmed it. This elusive subatomic particle forms a field that permeates the entire universe, creating the masses of the elementary particles that are the basic building blocks of everything in the known world--from viruses to elephants, from atoms to quasars. Starting where Nobel Laureate Leon Lederman's bestseller The God Particle left off, this incisive new book explains what's next. Lederman and Hill discuss key questions that will occupy physicists for years to come:* Why were scientists convinced that something like the "God Particle" had to exist?* What new particles, forces, and laws of physics lie beyond the "God Particle"?* What powerful new accelerators are now needed for the US to recapture a leadership role in science and to reach "beyond the God Particle," such as Fermilab's planned Project-X and the Muon Collider? Using thoughtful, witty, everyday language, the authors show how all of these intriguing questions are leading scientists ever deeper into the fabric of nature. Readers of The God Particle will not want to miss this important sequel.
Product Details
ISBN-13: | 9781493086993 |
---|---|
Publisher: | Rowman & Littlefield Publishers, Inc. |
Publication date: | 08/06/2024 |
Sold by: | Barnes & Noble |
Format: | eBook |
Pages: | 336 |
File size: | 3 MB |
About the Author
Leon M. Lederman, Nobel Laureate was the author of the highly acclaimed Quantum Physics for Poets and Symmetry and the Beautiful Universe (both coauthored with Christopher T. Hill), as well as The God Particle (with Dick Teresi). He had served as the editor of Portraits of Great American Scientists and a contributor to Science Literacy for the Twenty-First Century. He was formerly the Resident Scholar at the Illinois Mathematics and Science Academy and Pritzker Professor of Science at the Illinois Institute of Technology, and he was director emeritus of Fermi National Accelerator Laboratory.
Christopher T. Hill, PhD is the coauthor with Leon M. Lederman of Quantum Physics for Poets and Symmetry and the Beautiful Universe. He is a theoretical physicist (Scientist III) and the former head of Theoretical Physics at Fermi National Accelerator Laboratory.
Read an Excerpt
Chapter 2
A Brief History of the Big Questions
The most fundamental of questions we are asking today concern the smallest objects, objects that lie far beyond the atom, the quarks,
the leptons (“matter”) and gauge bosons (“force carriers”), the
Higgs boson, and whatever lies beyond these things. Here we are exploring a strange new world— world of the smallest things. No one has ever been here before, to examine what is happening at the smallest distances that are now probed by the Large Hadron Collider (LHC). This is not entirely blind exploration, for we actually have an inkling of what we are trying to understand—ut surprises may be around the next corner.
In short: we are attempting to answer the vexing question: What is the origin of mass? Mass is one of the most important defining quantities of matter. But where does it come from? What makes mass happen? Will we ever become skillful enough to calculate the mass of the electron or the muon or the top quark from a “first principle”? What shapes and controls and sculpts the elementary constituents of matter and their masses?
This is a bit like trying to answer the deep biological question “What and where is the genetic code of life?” The answer to that question came in the 1950s—it turned out to be encoded into a very long and durable molecule called DNA. And from that has come an entirely new set of capabilities,
as DNA can be “read” and “reread” and, eventually, we think, “rewritten.”
All structure and function and ultimately all diseases of living organisms are controlled by DNA and its associated processes. Understanding DNA
and its evolution is the foundation of understanding all life on Earth. Our open physics questions today are much like the biological ones before the
1950s: “What causes the phenomenon of mass?” Put another way, “What is the DNA of matter itself?”
To get some insight into the process of the exploration of nature, let us ask, what deep questions were our ancient ancestors asking over the past three millennia? Like newborn babies, our ancient ancestors awoke with rational minds and conscious awareness into a world with a “reality” of its own. It was difficult initially for them to shake off primitive prejudices, notions and fears,
unwarranted or otherwise, about things that seemed to happen or were only imagined to happen. There was an internal reality to the human mind in the early dawn of intelligence, voices that spoke in the night, apparent demigods lurking behind every tree, making all things, good or bad, happen. This led to peculiar notions, for example, that one must dance in strangely ostentatious ways, while wearing bizarre make-up and costumes, in order to make good things happen, perhaps to make it rain. Indeed, most appeals for divine intervention are just a variation on a rain dance and are motivated by something like the mortal fear of crop failure. It was difficult to discard that and to create a distilled “objective reality.”
But gradually there emerged a coherent understanding and philosophy of objective reality. Questions could now be posed and answers sought without reference to mythical beings and magic, without the fear of offending the particular gods that brought the rains. One learned to do
“experiments.” And one learned that the reproducibility of an experimental result was far more important than the mere opinions of the witch doctors and high priests. Does it really rain when we put on our costumes and dance about? No. But there are certain crops that can grow better in a dry climate than others, and certain clever ways to grow them. At some point the issue of understanding reality became “science.”
Eventually people asked the deeper questions: “What are all things made of?” “What are their properties?” “How do they interact with one another?” “What are the fundamental laws of nature that govern these objects?” These are practical questions, but they are also the biggest questions.
They deal with profound issues: “What constitutes physical reality?”
and “What is the nature of physical substance?” and “What is physical force, motion, space, and time?” The answers hold deep secrets, and perhaps the key to a better fire, a better sword, a cure for illness, perhaps a way to make the rains come or prevent them from leaving, or to make the best of what the conditions are, and how not to mess things up. By the end of the nineteenth century, here on Earth, the question: “What is the nature of matter?” was framed within the province of chemistry: All matter is formed from the basic atoms that comprise the chemical Periodic Table of the Elements—where “periodic”’ refers to their chemical properties.
The elements form chemical compounds and enter into chemical reactions according to specific empirical rules. The laws of physics are those of
Galileo and Newton, embellished by Maxwell, Gibbs, Boltzmann, etc.
Many thinkers from antiquity had previously developed a rudimentary concept of “elements.” These would be the basic, irreducible components out of which things are made. Among the earliest ideas were the so-called
“classic elements,” as described by Plato: “Air,” “Fire,” “Earth,” and “Water,”
as well as mysterious “Quintessence.” The latter was considered to be an all-universe-filling “ether.” This view of the nature of matter reduced every question to the five classic elements and offered a (very) tiny hint of an underlying order, but it certainly didn’t get into the details. It was more of a dismissive answer to questions about the inner nature of matter.
Other philosophers of antiquity, however, were actually quite modern from our perspective. The foremost of these was Democritus of Athens, one of most advanced thinkers in all of human history, considered by some to be the “father of modern science,” certainly the Galileo of his age. Democritus was born around 470 BCE, and died around 370 BCE, thus living to the ripe old age of about 100. He was often viewed as an eccentric fellow and largely ignored in his home town of Athens, and was supposedly detested by Plato, who denied ever meeting him (though this was unlikely since
Plato allegedly wanted all of Democritus’s books burned).
Democritus inherited the moniker “the laughing philosopher,” as he evidently found most of the ideas of other contemporary philosophers to be rather humorous, if not ridiculous. We can imagine him heckling Plato during a lecture in some curia, circa 400 BCE, perhaps asking a subtle and detailed question about a certain chemical reaction about which Plato could not begin to answer:
P: And the natural order and simplicity of nature is simply that all things can be resolved to the five “elements,” the “air,” the “fire,” the “water,”
the “earth,” and the “quintessence,” and that’s all of it.
D: Master, are these elements transmutable into one another?
P: No, truly not, sir, for as I say, they are elemental.
D: But of what element is the brilliant light of the sun?
P: (pause) I suppose . . . a form of quintessence as it does flow though space which is filled of quintessence and so it must be such.
D: And, master, of what element is papyrus?
P: Surely, papyrus is a form of the earth as it comes from the earth.
D: So, master, if I place a gem of spherically shaped quartz between the position of the sun, and that of a papyrus scroll, which you say is a form of the earth, I can direct, or “focus,” the sun-light, a form of quintessence, upon the papyrus and I can produce a fire. Have I not converted the quintessence into the fire or the earth into the fire?
P: I do not believe this can happen, sir.
D: I have set up the experiment here, master (Democritus directs Plato and the audience to a window at which he has an apparatus. With the apparatus he focuses sunlight onto a piece of scroll paper, and it shortly smokes then bursts into flames).
P: (impatiently) Well, if this is not a ruse then perhaps . . . perhaps light is really a form of fire, so you have not converted anything into anything else.
D: But if I should send the light, that you now say is fire, into an urn of oil, it becomes dark . . . where has the fire now gone? Has it become the oil which you would say is the earth?
P: Indeed . . . (pause, stammer) well, perhaps it is as we said quintessence . . .
D: Then as I burn the papyrus (the paper continues to smolder), which is a form of the earth, in the fire, and the smoke rises into the air, and the papyrus disappears, have I not converted the earth into air?
P: (long indignant pause)
D: Bbbbwwwaaahahahaha . . . (Democritus bursts into a sneering and callous laughter).
Democritus wanted real and detailed answers to scientific questions.
From Democritus we got a conceptual basis of the elements. These elements,
he reasoned, must have certain complex dynamical properties that cause them to ultimately shape and define the behavior of matter. The multitude of various properties of ordinary matter are reduced to the more fundamental properties of atoms. Some elements were envisioned to be little spherical balls that could freely flow (e.g., liquids), while others had hooks and could form stiff structural bonds (metals), and still others had block-like shapes that might make regular crystalline arrays (diamond or quartz). The theory had to explain all known phenomena correctly, perhaps even predict new observable phenomena, the standard to which science holds all theories.
Of course, this was an ultra-ambitious undertaking in those days.
Democritus had no microscopes, or particle accelerators, to test and validate his hypothesis. But his reductionist hypothesis implied rules and organizing principles for chemistry. Democritus dubbed the basic constituents of matter “atoms” from the Greek atmos (indivisible). Out of these basic building blocks we can construct more complex objects and the forms and shapes of all that we see. The behavior of the large-scale physical world is thus emergent from the fundamental properties of atoms.
This is a wholly modern view of the physical world, as well as one of the tasks of science. While, in Democritus’s theory, certain materials could change and rearrange their structure under chemical reactions (e.g.,
burning them, letting them rot, or dissolving them in water), the underlying atoms were immutable, unchangeable, invariant. His theory was useful and offered a prescription for further research. Here was the basic tenet of “fundamental particles,” and their role as the irreducible components of all things throughout the universe, which sculpt and shape the world through their own intrinsic properties.
Alchemists over the subsequent centuries went to work. They never succeeded in turning the element lead (Pb) into the element gold (Au),
or achieving any other elemental transmutation, for that matter. In countless attempts to do so they merely rearranged elements within the many exotic compounds, but they provided the service of amassing an enormous empirical “database” of recipes and processes and properties of chemicals that formed the foundation of the science of chemistry. In this sense,
Democritus’s theory was tested, found to be correct, but has been so significantly enlarged in detail by later science that it ultimately proved to be more of a philosophy, a prescription to actually do the hard work of science, and not to be merely contented with a dismissive shake of the wrist, invoking “air,” “fire,” “earth,” “water,” and “quintessence,” panacea for lack of a deeper understanding.
What we come into contact with on a daily basis, the “everyday matter,”
is the first layer of the “onion of nature.” It is comprised of “molecules,” which are either large or small groupings of atoms. Salt (NaCl), water (H2O),
oxygen in the air we breathe (O2), and methane (CH4), the gas we use to heat our homes, etc., are all molecules, composed of combinations of the more fundamental elements or atoms. Molecules can be broken down chemically into their constituent atoms, which can then be rearranged into other molecules. Just light a match to a certain mixture of oxygen molecules and methane molecules, and these will rapidly rearrange to form water molecules and carbon dioxide molecules, releasing a lot of heat.2 On the other hand,
sodium (Na), chlorine (Cl), hydrogen (H), oxygen (O), carbon (C), and so on, are all atoms, or “elements.” These are invariant, or unchanging, in chemical reactions—hey are the “fundamental particles” of chemistry.
The total numbers of these atoms never change in chemical reactions—the atom of gold (Au) cannot be changed into lead (Pb) by chemical reactions.
The atoms cannot be further subdivided without doing things that aren’t possible in a high school chemistry lab. To smash atoms apart, into
“smithereens,” takes us beyond the realm of chemistry. It takes us into a deeper layer of the onion of nature, the realm of atomic and nuclear physics,
eventually into the realm of quarks, leptons, and gauge bosons. These are,
today, the true “fundamental particles” of nature, perhaps to be replaced by smithereens in some science of the future.
By the mid-nineteenth century, based upon the accumulated knowledge of all the known chemical processes, the elements, or atoms, were classified according to their properties by the great Russian scientist
Dmitri Mendeleev. This classification scheme is called the Periodic Table of the Elements. The Periodic Table was a stunning summary of the thousands of years of alchemy, chemical science, and simply messing around with matter. It represented the reduction of the virtual infinity of molecules into a simple list of approximately 100 atoms found in nature (slightly more than 100 atoms is today’s count; it was significantly fewer at the time of Mendeleev; many elements, such as helium, were discovered later, and many of the heaviest elements are so radioactively unstable that they must be artificially produced in particle accelerators and are not to be found on our old high school chemistry classroom wall charts). The Periodic Table represented a pattern of repetitive chemical behavior in the properties and forms of atoms as one goes to heavier and heavier atoms. By its complexity,
however, it suggested that atoms may, themselves, be further reduced and may have internal structure, and that a deeper layer of subatomic matter must exist.
The “Physic is as an Onion” Metaphor
Mendeleev’s table was the beginning of the modern era of the science of matter.
To understand this, one must appreciate that nature is, empirically, organized much like an onion. Nature has different layers of phenomena and structures as one descends to smaller and smaller distance scales. And, going downward to shorter distances, we discover, is equivalent to going to higher and higher energy scales (higher “energy per particle”; we’ll define this more carefully momentarily). Although all of nature is governed by the same underlying fundamental laws of physics, the structures of complexity that we see in nature seemingly occur at different “strata” of phenomena, like an onion, and each stratum of nature is characterized by the energy needed to probe it.
What do we mean by “the energy needed to probe it”? We have to get a little bit technical here and introduce you to the common currency in talking about energies of fundamental particles and atoms: the electron volt or “eV.”4
Most of the science of chemistry, that is, the amount of energy involved in most chemical reactions, lies in the range from about 0.1 to 10 eV per atom.
This means that when a given atom enters into a chemical bond with another atom (or an existing molecule) to form a new molecule, roughly 0.1 to 10 eV
of energy is released. This is energy that comes from the forces that produce a chemical bond between two atoms, and it is typically released in the form of light, or the energy of motion, called kinetic energy.
The released energy is usually converted into heat (which is the aggregate random motion of atoms in a material), but it can also be seen as the light emitted from a fire or heard as the boom from a firecracker. You can usually see the released chemical energy with your eyes because a single visible particle of light, the photon, carries about 2 to 3 eV of energy—after all, the light entering our visual system that allows us to see is processed by various chemical reactions in our eyeballs and our brain, and so the perception of light entirely happens at the chemical energy scale.
If we can probe molecules with a source of energy of about 0.1 to 10
eV, we can often cause a chemical reaction to occur. For example, striking a match in a mixture of methane (CH4) and oxygen (O2) will provoke a rapid chemical reaction—a flame—yielding carbon dioxide (CO2) and water
(H2O). The match is generating photons and kinetic energy of motion of atoms of about an eV each from its own burning (usually oxygen combining with phosphorous). These energetic particles strike the methane and oxygen and nudge them into reacting, which emits more photons.
Then more and more energy is released in a chemical chain reaction, and
VAVOOM, you might have an explosion.
The physics, that is, the motion and interactions of electrons and atoms in chemical reactions—the stratum of the chemical reactions—is very much independent of, or decoupled from, what is going on in other stratums of nature. For example, to analyze everyday chemical phenomena,
one need not be bothered by such complications as the detailed motion of the protons and neutrons that comprise the inner atomic nucleus and that exist on much smaller distance scales than the overall size of the atom.
Nuclear physics is a far different energy stratum, measured in millions of electron volts, or “MeV” (see note 4), compared to the lowly 0.1 to 10 eV
stratum of chemistry. Nor need we, in studying chemistry, be bothered by the slow, lugubrious astronomical motion of the earth in its orbit around the sun. In fact, it is the relative motion of atoms and the electrons within the atoms that matters for chemistry. Thermal effects, the random motion and collisions of atoms due to heat, are typically about 0.1 eV per atom at room temperature, and they increase with temperature and therefore do have effects on chemical reactions (i.e., “cooking”). But the motion of the earth in its orbit is a common, uniform drift of the assemblage of all of the earth’s atoms, producing no high-energy inter-atomic collisions. Of course, if an asteroid collides with the earth, the relative motion of the asteroid’s atoms hitting the earth’s atoms involves very high energies, and very serious chemistry, even nuclear physics, will occur!
While the triumph of Mendeleev’s Periodic Table of the Elements formed a basis for understanding all chemical reactions, we learned in the early twentieth century that the atoms themselves are not truly elementary:
they are composed of even smaller, more basic objects. To understand this we must probe into the atom. And, as it goes with probes, the probe we use to analyze something should preferably be smaller than the object we wish to probe. If the probe is bigger than the object probed, it becomes a bludgeon or battle-ax, and battle-axes don’t work so well for dissecting tiny things or performing dental surgery, etc.
A simple home-brew experiment that you can perform, e.g., at a child’s birthday party, will illustrate this point.
A Simple Home-Brew Experiment
Get a beach ball and a straw. Have someone blindfold you. Have your assistant take some randomly chosen small items unknown to you, like a peanut, an acorn, a coin, nuts and bolts, a few other small things, and place them on a table in front of you. Now, while still blindfolded, take hold of the beach ball with both hands. Holding only the ball, try to use it gently as a probe of the small objects on the table, the peanut, the acorn, etc. Can you discern which little object is which, while blindfolded, and coming into contact with them only through the very large beach ball? We would guess not, unless you peeked.
Next, take one end of the long straw and, while still blindfolded, use it to trace out the forms of the same small objects. Can you now discern what these objects are and which is which? When you trace out the objects’
shapes you must use a little thought and a little imagination to try to figure out what each of them is—ou’ve become both an experimentalist and a theoretical physicist at the same time—ike Enrico Fermi. With enough effort and thought you can probably figure out what little objects were placed before you on the table. Perhaps you can tell a dime from a nickel,
and chunk of cauliflower from a golf ball. Go ahead—ry it!
One thing is clear, if not obvious, from this experiment: a probe that is many times larger than the object to be probed does not work very well. Holding the beach ball, we doubt you can discern a nickel from a dime, if you can even detect either of them. On the other hand, probes that are much smaller than the object itself allow us to readily resolve the object’s structure—ven without seeing it with our eyes. This simple principle holds true in all of the strata of the onion of nature, including the stratum of subatomic particles.
To explore the structure of the unknown “something,” we must construct a probe that is smaller than the “something” we seek to study.
This seems at first blush to pose what appears to be an insurmountable barrier to studying small objects, like the innards of an atom or a particle inside an atom. How can we study a particle’s inner structure if all we have are other particles of the same size? Ah-ha! Here is where two of the greatest revolutions of science come to our aid: the quantum theory and
Einstein’s theory of relativity.
Essentially, we learn from quantum theory that all particles in nature are also waves. This seems to be a ridiculous and nonsensical paradox, but it is the mysterious and jarring reality of quantum theory. The effects of waves vs. particles for most things don’t show up until we reach atomic dimensions,
but they can be seen readily for ordinary light. But to be precise, a quantum state is neither a particle nor a wave—t is both at once!
Small objects can be described by quantum mechanical waves that are associated with the probability of detecting a point-like particle at any point in space and time. That is a mouthful, and the interested reader should grab a copy of our book Quantum Physics for Poets (Amherst, NY: Prometheus
Books, 2011). However, if you can just “ride the wave” with us for a few more paragraphs, you need only accept that a wave always has a characteristic wavelength. The wavelength is just the familiar distance between two crests or two troughs of a wave, like a water wave. It is the quantum wavelength that tells us how big an object is when it used as a probe.
Now here is a second relevant fact about quantum physics: as we increase the energy of any particle, its quantum wavelength becomes smaller and smaller. When the wave motion approaches the speed of light, then Einstein’s theory of relativity kicks in. If you double the energy of a particle moving near the speed of light, you will halve its quantum wavelength. So, investing a lot of energy in a particle makes its quantum wavelength smaller. This,
in principle, allows us to make an arbitrarily tiny probe simply by accelerating a particle to arbitrarily high energies. This is the most important principle underlying microscopes and particle accelerators. The more energy in a particle, the smaller it becomes. And, by the way, you now understand why today’s particle accelerators are very large: it takes a very large accelerator to put a lot of energy into a particle to make it become a smaller probe.
The wavelength of ordinary visible light ranges from, approximately,
higher-energy blue light, 0.00004 centimeters (4 × l0-5 cm, about 3 eV per photon; recall that a centimeter is about a half an inch) to lower-energy red light, 0.00007 centimeters (7 × l0-5 cm; about 2 eV per photon). A
typical visible particle of a light, a photon, has a quantum wavelength in this range, with an energy of approximately 2 to 3 eV. Objects larger than about 0.0001 centimeters (l0-4 cm) can be readily probed with visible light because they are smaller than the wavelength of the light wave. You need only make a precise optical microscope to do this, and you can see little things that your eye cannot resolve.
However, visible light falters when it is used to study structures smaller than this size scale, such as the tiny components found inside the living cell of a biological organism. Visible light is unable to resolve two objects of much less than 0.00001 centimeters (l0-5 cm) or smaller. You now know the reason: these objects are smaller than the wavelength of the visible photons of light—visible photons are as useless as beach balls to probe such small distance scales. No improvement in your microscope optics can ever improve the image. You could spend hundreds of thousands of dollars on a top-of-the-line Bausch and Lomb microscope, and still the tiniest denizens deep inside living organisms will only appear fuzzy or will not appear at all.
Crank up the magnifying power of your microscope, and all you’ll get is a bigger fuzzier image. You absolutely cannot see DNA in an optical (lightbased)
microscope. Visible light is hopeless to use as a probe of an atom at the atomic-size scale of 0.00000001 centimeter (10-8 cm) or less.
Fortunately, at shorter distance scales, even down to the atomic stratum and beyond, electrons become excellent probes. Electrons can be accelerated in a small type of particle accelerator called an electron microscope,
giving them more energy. Electrons, too, have a quantum wavelength, as do all particles. Electrons can easily be endowed with kinetic energies of about 20,000 eV (that’s 10,000 times more than a visible photon). This is the energy of acceleration of the electrons in the old TV picture tubes that could at one time have been found in any household but that have now gone the way of the horse and buggy. At this energy the electrons have a quantum wavelength of about 0.000000001 centimeters (10-9 cm), considerably smaller than that of visible light, and they can be used to make images of DNA, a virus, and even resolve individual atoms.
Peering Inside the Atom
The first peek into the internal structure of the atom, the “atomic onion layer of nature,” began about 50 years after Mendeleev with a British scientist named J. J. Thomson.6 Thomson cleverly demonstrated in 1897
that certain “rays” that could be provoked out of atoms in an electric discharge tube (something like a fluorescent light tube) were actually particles. In particular, Thompson established that these particles lurked within all atoms, and he called the new particle the electron. Thomson is deservedly heralded as the “father of modern particle physics” for this discovery. He proved that electrons were extremely low-mass particles,
weighing two thousand times less than the atom itself, and that they each carried a negative electric charge.
Thanks to the work of J. J. Thomson, atoms were now known to be full of these very lightweight, negatively charged electrons. But atoms themselves are normally electrically neutral, i.e., they have no electric charge;
they can be “ionized” and lose an electron, hence they acquire the opposite,
positive charge. Obviously, then, there would have to be an equal amount of positive electric charge inside the atom, balancing the negative electric charges of the electrons. But where this positive charge resided within the atom remained a mystery. In 1905, Thomson had proposed a theoretical model of the atom in which the positive electric charge is a medium that is uniformly dispersed throughout the entire atom, with the electrons embedded within it like raisins in a loaf of raisin bread.
Throughout this era a gruff walrus of a young man, Ernest Rutherford,
played a prominent role in unraveling the inner properties of matter.
Rutherford was a skilled and masterful craftsman and won the Nobel
Prize for his work in elaborating the properties of radioactivity (which we’ll describe later), and he had now become the director of the famous
Cavendish Laboratory in Cambridge, England.
Rutherford had grown up as one of a dozen children in a farm family in rough-and-tumble New Zealand, where he had learned hard work, thrift,
and tinkering with technological innovation. As a child he’d played with clocks and made models of his father’s waterwheels, and by the time he was a graduate student he was investigating the physics of electromagnetism.
He had managed to devise a detector of wireless (radio) signals even before
Marconi began his famous experiments that led to the wireless telegraph.
When a scholarship brought Rutherford to the Cavendish Laboratory, he hauled his wireless device along to England and was soon sending and receiving signals over half a mile, a feat that impressed the Cambridge dons, including J. J. Thomson, the Cavendish director at the time. Later
Thomson would declare, “I have never had a student with more enthusiasm or ability for original research than Mr. Rutherford.”
By 1909, Rutherford and his students were shooting tiny subatomic probes, called “alpha particles,” at a piece of thin gold foil. They were carefully measuring the way in which the particles were slightly deflected as they scattered off of the heavy gold atoms in the target foil. The alpha particles were produced from the radioactive decay of the element radium,
which naturally accelerated them to high energies—here were no manmade particle accelerators in those days. Alpha particles were now known,
thanks to Rutherford, to be very heavy compared to an electron, and with a little energy they have a very tiny quantum wavelength and are therefore capable of probing deep inside the atom.
One day something utterly unexpected happened. The alpha particles were usually deflected only slightly by their passage through the gold foil,
and the scientists were measuring in detail this “forward scattering.” They decided, simply as a sanity check, to see if there was any “backward scattering.”
To their astonishment they found that one in 8,000 alpha particles bounced back toward the source. As Rutherford remembered it, “It was as if you fired a fifteen inch artillery shell at a piece of tissue paper and the shell came back and hit you.”9 What was happening here? What kind of thing inside the atom was repelling the positively charged and massive alpha particle?
No one before Rutherford had devised any way of mapping the inner shape of the atom. According to the “raisin bread” model of the atom of J. J.
Thomson, the alpha particles should always have bullied their way straight through the atom—lways! The atom was like a big glob of shaving cream,
and the alpha particles were rifle bullets. Rifle bullets would tear straight through globs of shaving cream. Imagine seeing a rifle bullet occasionally deflected and ricocheting backward upon colliding with a blob of shaving cream. Such was the observation of Rutherford and his students.
Rutherford devoted his full energies to understanding this remarkable discovery. According to his detailed calculations there was only one way that any alpha particles could ever be deflected backward. That could only happen if the entire mass, and positive charge, of the atom was concentrated into a tiny volume in the center of the atom—he “atomic nucleus”
was discovered! The nucleus’s hefty mass and large positive charge could repel the positively charged alpha particles that came within its range and deflect them through a large angle, even kick them backward. It was as if within the glob of shaving cream there were dense, hard ball bearings that could cause bullets to collide and deflect. The electrons were orbiting this dense central charge of the atom. The raisin bread theory of the atom of J. J.
Thomson was tossed in the trash-bin. An atom now resembled a tiny solar system, with miniature “planets” (electrons) orbiting a dense “dark star” at the center (nucleus), and it was all held together by electromagnetism.
Further experiments indicated that the nucleus was indeed tiny—netrillionth of the volume of the atom—ven though most of the mass of the atom, more than 99.98 percent of it, resided in the nucleus. At the time of this discovery, within this tiny solar system model of an atom, all the classical laws of physics were still thought to be rock solid, just as in the macroscopic solar system with the sun and its planets. The same laws of classical physics were believed to work in the atom just as they did everywhere else—until Niels Bohr showed up.
Thinking through the Atom
Niels Bohr was a young theoretical physicist from Denmark who was studying at the Cavendish Lab, and he happened to attend a lecture by
Rutherford. He was immediately captivated by this new atomic theory of electrons orbiting nuclei. Bohr arranged to visit the great man for four months in 1912, while Rutherford, at the time, was working in Manchester.
Sitting down and thinking about the new data, Bohr quickly perceived something profoundly significant about Rutherford’s planetary model of the atom. It was a complete disaster, according to the known laws of physics!
Bohr realized that, in their state of rapid circular motion about the nucleus, electrons would radiate away all of their energy in the form of electromagnetic waves very quickly. Like the swoop of a seagull into the sea, the electron orbits would quickly shrink to zero, within a tenth of a millionth of a billionth of a second. The electrons would spiral down into the nucleus. This would make the atom, ergo all of matter, chemically dead and the physical world as we know it impossible. The exact classical equations of electricity, due to Maxwell and based upon Newtonian physics,
spelled disaster for the atom. Either the model had to be wrong, or the venerable laws of classical physics had to be wrong.
Bohr applied himself to understanding the simplest atom—the hydrogen atom—which would have a single electron in orbit around a positively charged nucleus consisting of a single positively charged particle called a proton. Thinking about the new quantum ideas that were in the air, that particles are also waves, young Bohr was led to propose a very novel idea. He argued that only certain special orbits can ever happen for electrons in atoms because the motion of electrons in these orbits must be like that of waves.
These are like the natural wavelike motion of a ringing bell or a Chinese gong, with a dominant lowest tone, or mode, and a sequence of “overtones”
or “harmonics.” The lowest mode, what we mostly hear when the bell tolls,
would be the one with the least amount of energy, corresponding to the wave motion where the electron is moving closest to the nucleus. In this lowest orbit the electron cannot radiate away anymore energy, because this is the state of lowest possible energy for the electron motion—he electron has no lower energy state into which to go. This special orbit is called the ground state.
This is one of the hallmarks of quantum theory: atoms cannot just collapse into nothingness and are actually supported by the quantum wave motion,
leading to the existence of a ground state, the state of lowest possible energy.
In three papers published in 1913, Bohr articulated his audacious quantum theory of the hydrogen atom. Each of the atom’s magic harmonic orbits is characterized by a certain energy. An electron emits a definite amount of radiation when it “jumps” from an orbit of higher energy down to one of lower energy. It emits a photon, the particle of light, whose energy is given by the difference of the energy of the two orbits. With billions of atoms doing this at the same time, we see bright and unique colors for the emitted light, the photons all having exactly the same energies.
Bohr put his theory to work and calculated the wavelengths of all the emitted photons, the colorful “spectral lines,” seen in a spectroscope from hot glowing hydrogen gas. His formula worked perfectly! Electrons now moved in “Bohr orbits,” or “orbitals,” within the atoms.
None of this made the slightest sense in the familiar framework of
Newtonian-Galilean physics. It required sweeping changes in our understanding of physics and the further development of the radical new ideas of quantum mechanics. In any case, atoms were indeed seen, by now, to be made of still smaller objects: the electrons and the atomic nucleus, and the rules of motion, the relevant laws of physics, were now totally new and totally quantum mechanical.
Quantum Waves and Cosmic Rays
The quantum wavelike behavior of all matter was established within the first several decades of the twentieth century through numerous experiments, and the quantum theory was cobbled together (see note
3). By accelerating particles, we can shrink their quantum wavelengths.
The overall characteristic “size of the atom” is determined by the “Bohr radius,” which is the size of the quantum wave of the electron ground state of hydrogen and is about 0.000000005 centimeters (0.5 × 10–8 cm). The atomic nucleus is very much smaller, about one-hundred-thousandth the size of the Bohr radius. To explore these much smaller distances required much more energetic probes. High-energy particle accelerators would not arrive on the scene until the 1950s.
Nature, however, provides one extremely high-energy source of particles.
These are very energetic cosmic rays that bombard Earth coming from deep outer space.11 The cosmic rays are produced by violent processes in distant star systems, such as supernovae, pulsars, and active galactic nuclei.
They are steered on the voyage to Earth by galactic magnetic fields, and their sources generally cannot be identified. The energies of cosmic rays extend way up beyond the highest energies of any particles we have ever seen or produced in the laboratory, higher than the LHC itself. The highest-energy cosmic rays ever detected have about 100,000,000,000,000,000,000 electron volts of energy (That’s 1020 eV; for comparison the LHC design energy is 14 trillion eV, or 1.4 × 1013 eV), but these cosmic rays are extremely rare,
only one of them passing through one square mile of sky every century!
However, cosmic rays of energies up to about 1,000,000,000,000 eV (1,000
GeV; that’s 1,000 Giga-eV, and 1 Giga = 1 billion) are sufficiently abundant that they can be put to good use to act as scientific probes—ven to discover new particles.
Typically, cosmic rays, mostly protons and some heavy atomic nuclei,
collide with the nuclei of nitrogen and oxygen high up in the earth’s atmosphere,
perhaps 10 to 20 miles up. These collisions smash the nuclei apart and send other particles as debris downward, into the atmosphere. This typically generates a plume of electrons from all of the subsequent ionization and more collisions of debris particles with other atoms. Long-term exposure to this radiation would be harmful, but we are protected by the atmosphere at ground level. Occasionally some new particle could, in principle,
be produced, and it might be detected all the way down at the surface of the earth, provided it is a deeply penetrating particle. Some experiments place detectors high up on mountaintops or in balloons to try to detect less deeply penetrating particles.
From the 1930s, and even beyond the 1950s, when particle accelerators finally became available, most of the early discoveries of new elementary particles came from cosmic ray experiments. And, to this day, cosmic rays continue to serve us well in providing information that is hard to obtain from accelerators. Most recently, the masses of neutrinos were established using cosmic rays as neutrino sources in 1995 (see chapter 10).
The energy stratum of the nucleus is measured in terms of millions to hundreds of millions of electron volts. To unravel the nucleus required probes of hundreds of millions of electron volts, so people in the 1930s and
1940s turned to exploit cosmic rays.
The Mystery Deepens: What Holds the Atomic Nucleus Together?
By the mid-1930s, building upon Rutherford’s discovery and the new quantum theory, it was realized that the atomic nucleus is composed of particles called protons and neutrons. The proton and neutron are very similar particles, each having about the same mass, but there is a big difference: the proton has a positive electric charge, while the neutron is electrically neutral. Hydrogen has the simplest nucleus that consists of a single proton, but all heavier nuclei are made of combinations of protons and neutrons, just as molecules are made of atoms. For example, the normal helium nucleus contains two protons and two neutrons.
Nuclei, like helium containing two or more protons, can only be held together by a very strong force—which is simply called the strong force. This nuclear-binding strong force has to be ultra-strong because the protons each have positive electric charges and therefore repel one another electrically.
The nucleus of an atom like helium would instantly fly apart unless an overwhelmingly strong force compensated for this electrical repulsion and bound the protons, together with the neutrons, into the compact nucleus. Indeed, a nucleus like uranium, with 92 protons, is very unstable because of the enormous repulsive electrical forces of so many protons.
Uranium therefore has many isotopes, such as U233 (where 233 = 92 protons
+ 141 neutrons), U235 (92 protons + 143 neutrons), U238 (92 protons + 146
neutrons), etc. Notice that we can package more neutrons into uranium because they are electrically neutral, and even help the binding together of the 92 protons. The strong force is about 10,000 times stronger than electromagnetism, and it can hold nuclei together up to about 100 protons.
Very heavy nuclei with lots of protons are generally unstable due to this electric repulsion. They undergo fission (spontaneously break apart)
into lighter nuclei.
Forces, in our quantum world, are actually generated by particles. The force between two objects, like a proton and a proton, is caused by lighter particles that jump to and fro between the two protons. The repulsive electrical force is caused by the jumping of photons, the particle of light, back and forth between the protons. The strong force had to be due to something else.
A particle responsible for the strong force was predicted by the Japanese physicist Hideki Yukawa,13 in 1935, based upon the known properties of the atomic nucleus. Yukawa reasoned that the force of electromagnetism is comparatively long range—the electric force between charged particles decreases “slowly,” by the inverse square law (it falls like 1/r2 where r is the distance between the two particles). This inverse square law arises because the photon is a massless particle and can easily jump between nearby or distant electric charges. The force of electromagnetism is also somewhat feeble, because the “jumping probability” in quantum theory involves a small number, essentially the (square of the) electric charge (see the
Appendix). This gives rise to the electric force that binds electrons to the positively charged protons in the nucleus.
On the other hand, an atomic nucleus is very small, a typical radius of about 0.0000000000001 centimeters (10-13 cm), about one hundred thousand times smaller than the electronic orbits that define the chemical size of the atom. This arises in part because of the much larger masses of protons and neutrons than electrons, but also by the strength of the strong force that overcomes the electric repulsion between protons. Furthermore,
the nucleus is quite compact, requiring that the particle of the strong force need not produce a long-range or inverse square law force (which would have been detected outside the nucleus), but rather it is a short-range force.
Yukawa realized that this required a new particle that could hop back and forth between protons and neutrons, causing the strong force, and the new particle would need to have a mass of about 100,000,000 eV (100 million eV, or 100 MeV; see note 4) to account for the short range of the new force.
Figure 2.2. Forces Arise as the “Exchange of Particles.” The force between two particles arises from the “exchange of particles.” Two electrons, or any electrically charged particles,
interact by exchanging photons, which are the particles of light.
A proton and neutron strongly interact by exchanging pions.
This is a tall order, but it certainly pointed the particle searchers in the right direction. And remarkably, in 1936 a particle with a mass of 100 MeV,
called the muon (pronounced mew-on), or m, was discovered in 1937. It seemed to be the thing predicted by Yukawa, but people soon realized that the muon was a case of false identity—the cops had arrested the wrong guy.
The muon was discovered by using cosmic rays that (somehow) produced it
10 miles up in the sky. The muons then traveled to the surface of the earth where they could be detected. The reason the muon was initially thought to be the particle Yukawa had predicted (called the pion [pie-on], or p)
was because it had Yukawa’s predicted mass. But the muon did not interact strongly enough with protons and neutrons to be a pion since it could travel all the way to the earth’s surface (muons only interact electromagnetically,
or through the weak force). This new particle definitely was not the agent of the strong force, as predicted by Yukawa. In fact, the muon seemed to be a mere carbon copy of the electron but 200 times heavier, with a lifetime of about 2 millionths of a second (whereby the muon “decays” into an electron and a pair of neutrinos).
Almost all pure physics research was interrupted by World War II, as the world’s scientists were redirected to serve military needs. The quest for
Yukawa’s pions could resume only after the war. In the meantime humans had conquered the atomic nucleus, with its strong force—nd unleashed its fury.
Table of Contents
Acknowledgments 9
Chapter 1 Introduction 11
Chapter 2 A Brief History of the Big Questions 35
Chapter 3 Who Ordered That? 57
Chapter 4 All About Mass 81
Chapter 5 Mass under the Microscope 93
Chapter 6 The Weak Interactions and the Higgs Boson 127
Chapter 7 Microscopes to Particle Accelerators 149
Chapter 8 The World's Most Powerful Particle Accelerators 169
Chapter 9 Rare Processes 193
Chapter 10 Neutrinos 217
Chapter 11 Project X 229
Chapter 12 Beyond the Higgs Boson 243
Appendix 253
Notes 279
Index 319